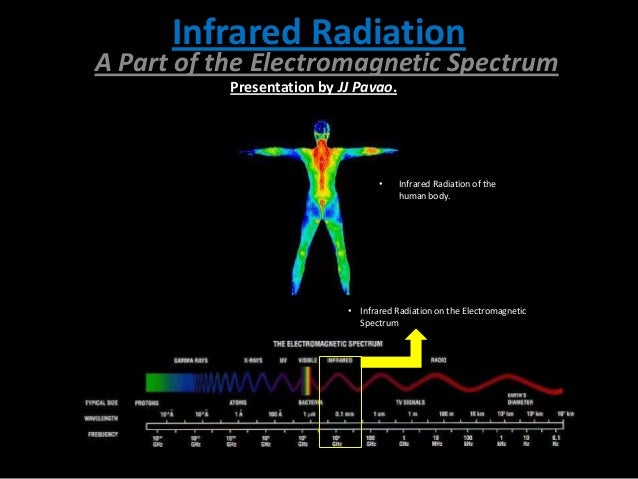
Biological effects and medical applications of infrared radiation
#WakeUp
Shang-Ru Tsai, PhD1,2 and Michael R Hamblin, PhD2,3,4,*
The publisher's final edited version of this article is available at J Photochem Photobiol B
See other articles in PMC that cite the published article.
Abstract
Infrared (IR) radiation is electromagnetic radiation with wavelengths between 760 nm and 100,000 nm. Low-level light therapy (LLLT) or photobiomodulation (PBM) therapy generally employs light at red and near-infrared wavelengths (600–100 nm) to modulate biological activity. Many factors, conditions, and parameters influence the therapeutic effects of IR, including fluence, irradiance, treatment timing and repetition, pulsing, and wavelength. Increasing evidence suggests that IR can carry out photostimulation and photobiomodulation effects particularly benefiting neural stimulation, wound healing, and cancer treatment. Nerve cells respond particularly well to IR, which has been proposed for a range of neurostimulation and neuromodulation applications, and recent progress in neural stimulation and regeneration are discussed in this review.
The applications of IR therapy have moved on rapidly in recent years. For example, IR therapy has been developed that does not actually require an external power source, such as IR-emitting materials, and garments that can be powered by body heat alone. Another area of interest is the possible involvement of solar IR radiation in photoaging or photorejuvenation as opposites sides of the coin, and whether sunscreens should protect against solar IR? A better understanding of new developments and biological implications of IR could help us to improve therapeutic effectiveness or develop new methods of PBM using IR wavelengths.
Keywords: Infrared neural stimulation, photoaging, DNA damage, brain neuroprotection, ROS, ATP, water molecules, heating
1. Introduction
Infrared (IR) is a type of electromagnetic radiation, including wavelengths between the 780 nm to 1000 μm. IR is divided into different bands: Near-Infrared (NIR, 0.78~3.0 μm), Mid-Infrared (MIR, 3.0~50.0 μm) and Far-Infrared (FIR, 50.0~1000.0 μm) as defined in standard ISO 20473:2007 Optics and photonics -- Spectral bands [1]. Several studies have reported that IR can improve the healing of skin wounds, photoprevention, relieve pain, stiffness, fatigue of rheumatoid arthritis, ankylosing spondylitis, potentiate photodynamic therapy, treat ophthalmic, neurological, and psychiatric disorders, and stimulate the proliferation of mesenchymal and cardiac stem cells [1–9].
Low-level light therapy (LLLT) is defined as “Treatment using irradiation with light of low power intensity so that the effects are a response to the light and not due to heat. A variety of light sources, especially low-power lasers are used.” in the Medical Subject Headings (MeSH) Descriptor Data 2017. Photobiomodulation (PBM) therapy is “A form of light therapy that utilizes non-ionizing forms of light sources, including lasers, LEDs, and broadband light, in the visible and infrared spectrum. It is a nonthermal process involving endogenous chromophores eliciting photophysical (i.e., linear and nonlinear) and photochemical events at various biological scales. This process results in beneficial therapeutic outcomes including but not limited to the alleviation of pain or inflammation, immunomodulation, and pro-motion of wound healing and tissue regeneration.” as a defined in Anders et al. [10]. It is now agreed that “PBM therapy” is a more accurate and specific term for the therapeutic application of low-level light compared with “LLLT”.
All photobiological responses are determined by the absorption of energy by photoacceptor molecules (chromophores) during light irradiation. It is important to clarify the molecular mechanism of light interactions with tissue by identifying the photoacceptor molecules. IR-induced physiological effects are thought to be due to two main types of photoacceptor (i.e., cytochrome c oxidase and intracellular water) [11]. Photon absorption converts light into signals that can stimulate biological processes [12]. The action of IR light on water dynamics in membranes, mitochondria and/or cells could modulate signaling pathways, production of reactive oxygen species (ROS), ATP (adenosine triphosphate), Ca2+, NO, and inositol phosphates group [13–16]. Secondary effects are always preceded by primary effects, including stress signaling, metabolic processes, cytoskeleton organization, cell proliferation/differentiation, and homeostasis (depending on injury or metabolic redox potentials) [17, 18]. Additionally, Shapiro et al. demonstrated that IR light could excite cells through water absorption, with a temperature increase affecting the plasma membrane and altering the electrical capacitance, thereby depolarizing the target cells [19].
Pollack et al. have demonstrated that water in specific locations within cells exists as a more chemically/biologically active molecule [20]. Most intracellular water is dynamic and has an ordered structure to support the life processes in biological systems [21]. Since the water electromagnetic absorption spectrum is mainly in the IR region, photon absorption can result in a rapid increase in intracellular temperature [22], which may promote unwanted physiological changes in temperature, pH, osmosis, and ATP yield [23, 24].
For billons of years, the sun has generated IR radiation and living organisms on earth have evolved to deal with IR radiation as an important environmental factor depending on their habitat. Many ancient therapies have applied sunlight for wound healing and pain relief. The spectrum of sunlight in the environment and the corresponding water absorption spectrum are shown in Figure 1 [25]. It is clear that the solar emission and the strong water absorption bands are nearly matched. Before sunlight penetrates the atmosphere, it is presents a more uniform emission spectrum. While sunlight reaches the ground, some bands have been absorbed by environmental gas or water molecules in the atmosphere. Since the human body is made up of 70% water, it can potentially accumulate a large amount of energy that could modulate biological processes, by strong resonant absorption of IR radiation from sunlight mediated by water molecules.
Superposition of spectra of solar irradiance and water absorption showing that the most significant areas of overlap occur in the region of 800–1300 nm
In recent years, the combination of technical, clinical, and photobiological principles have become important to understand the therapeutic effects of LLLT. For example in recent years, optical fiber delivery systems have become an important technology to facilitate LLLT [26]. Fiber-optics can transmit light at specific wavelength over long distances by utilizing total internal reflection, allowing them to bend along its path and focus the emission spot on a specific area. Although the light delivery procedures needed for LLLT to be used for diseases of the lungs and airway are difficult, optical fibers inside needles can be applied [27].
In addition, non-invasive, long-range, energy delivery has been described using an infrared-pulsed laser device (IPLD) of 904 nm pulsed at 3 MHz, which was claimed to have an original mechanism of action termed “photo-infrared pulsed bio-modulation” (PIPBM). The device was applied in a clinical trial of advanced cancer patients and a case of age-related macular degeneration (geographic atrophy) with associated neurological disease, it demonstrated sufficient evidence for its selective, long-distance, reparative and/or regenerative physiological effects [16, 28, 29].
Previous clinical studies have shown that LLLT has a wide range of benefits on various patient populations, different medical indications and conditions without any serious risk of adverse effects. Adequate dosimetry is important for LLLT and PBM therapy; a basic principal has emerged called the “biphasic dose response”, where larger doses of light have been found to be less effective than smaller doses [30]. This phenomenon is seen in the beneficial neurological effects of transcranial LLLT for traumatic brain injury, where the results vary significantly depending on the number of treatments and the energy density of each individual treatment.
The present review paper will only summarize some of the key studies about the new application and scientific findings related to IR radiation. It will particularly focus on the new applications, including IR emitting materials for clothing, IR sauna therapy, Waon therapy etc. In addition, we present some newly-emerged scientific findings about neural stimulation, photoaging, photorejuvenation, antitumor action, neural and adipose regeneration.
2. New Development and Application of Infrared Therapy in Biological Fields
2.1. Infrared Emitting Materials for Clothing
In recent years nanotechnology development has provided functional sportswear with many properties to enhance sports activities, performance, efficiency and comfort. For example, sportswear should allow the wearer to stay warm in cold environment, and keep cool in hot situations through transferring sweat away from the skin. In general, the mechanism of action of IR radiating materials is to transform heat energy from the body (convection and conduction) into radiation within the IR wavelength range between 3~20 μm to induce homeostasis and photobiomodulation via deeper penetration of IR radiation and water molecule absorption in the skin [25]. The use of materials that generate IR radiation is possibly helpful to enhance blood circulation and the metabolism of human body.
Previous studies have found that the effects of IR can activate fibroblasts, increase more collagen synthesis and the expression of transforming growth factor-beta1 (TGF-beta1) in rat wounds [31]. Previous studies have found that the incorporation of nanoscale germanium (Ge) and silicon dioxide (SiO2) particles into composite fibers producing poly(vinyl alcohol) (PVA) nanofibers. The emission wavelength of these nanofiber membranes were in the range of 5–20 μm at 37 °C and exhibited an emissivity value of 0.891 (a perfect black body has a maximum emissivity of 1) and an emissive power of 3.44×102 Wm−2 with a web area density of 5.55 gm−2. Far-infrared radiation-induced antimicrobial properties can be effective for bacterial reduction against both Staphylococcus aureus and Escherichia coli at 99.9 %, and showed a reduction of Klebsiella pneumoniae by 34.8 % [32].
Soccer players have used FIR emitting clothing (density of 225 gm−2, 88% far IR radiation emitting polyamide 66 Emana yarn (PA66) fiber, 12% Spandex, emissivity of 0.88 and power emitted of 341 W/m2 at 37°C at 5–20 μm wavelength range). These garments were used for 10 hours as sleepwear over three successive nights to relieve delayed-onset muscle soreness at 48 h after an intense plyometric exercise session [33].
A far-infrared emitting plaster has been applied for the therapeutic management of knee osteoarthritis. The posterior surface of the patient’s knee was treated by applying the plaster for 12 h a day and for 5 days a week for a duration of treatment of 4 weeks. The plaster was manufactured by Chongqing Kaifeng Medical Instrument Co. Ltd, China, which provided a plate coated with a proprietary mineral formation consisting of 33 elements designated to generate far IR through the action of a radiator. The longitudinal view ultrasound scan of the anterior compartment of the knee was monitored in the study. It showed that the patients from the FIR group had less joint effusion (40%) compared to baseline (80%) [34].
Ting-Kai Leung et al. have employed a ceramic powder (manufactured by Bioenergy Development Ltd, Taoyuan, Taiwan) for in vitro and in vivo studies. Its average emissivity was 0.98 at wavelengths between 6–14 μm with non-thermal effects at room temperature. The experimental targets included MCF-7 breast cancer cells, macrophage cells, melanoma cells, myoblast cells, chondrosarcoma cell line, human breast epithelial MCF-10A cells, and knees of rabbits [35]. The most important research result was that this bioceramic preparation could relieve inflammatory arthritis of rabbit knee joints [36]. The rabbits received intra-articular injections of lipopolysaccharide (LPS) to induce sterile inflammation, and were then placed in a cage surrounded by bioceramic-containing layer in the treatment group. Positron mmission tomography (PET) scanning showed that the bioceramic was capable of relieving inflammation in the joints at 7 days after LPS injection.
2.2. Infrared Saunas and Waon Therapy
The use of far-infrared saunas for medical treatment is based on deep skin-penetration of the radiation to restore homeostasis of thermal regulation. In sedentary patients suffering from osteoarthritis or cardiovascular respiratory problems, far-infrared saunas could be applied as an alternative to moderate exercise. They produce therapeutic effects without any adverse effects on congestive heart failure, premature ventricular contractions, brain natriuretic peptide levels, vascular endothelial function, weight loss, oxidative stress, or chronic fatigue [37].
Waon therapy means the body is warned in an IR chamber for 15 min at 60°C, and then they are wrapped in thermal blankets and laid down to maintain heat for an additional 40 min, and finally patient drinks water to replenish moisture lost by perspiration. It can improve cardiac function and is useful in rehabilitation [38].
Waon therapy was performed once daily, 5 days each week for 2 weeks. There were a total of 76 Waon therapy patients and 73 control subjects studied at 19 sites [39]. The plasma B-type natriuretic peptide values, “New York Heart Association” disease classification, 6-min walking distance, and cardiothoracic ratio were significantly improved in the Waon therapy group compared to controls. The trial demonstrated safety and efficacy to manage this target population with chronic heart failure.
Waon therapy exhibits an adjuvant effect for chronic obstructive pulmonary disease. The Waon group showed larger vital capacity and peak expiratory flow than the control group. Further studies are needed to investigate the mechanism of action, specifically whether Waon therapy may be associated with increasing airway NO flux [40].
Chronic heart failure induces vascular endothelial dysfunction. It has been demonstrated that IR sauna therapy improves vascular endothelial dysfunction in hamsters with experimental cardiomyopathy, which were treated daily with an experimental far IR sauna system for 15 min. After 4 weeks, the mRNA for arterial endothelial nitric oxide (NO) synthase (eNOS) (as well as the protein expression), and NO production were increased significantly compared with normal controls [41].
3. Emerging Studies of Infrared Therapy
3.1. Neural Stimulation
Infrared neural stimulation (INS) has higher spatial resolution without electrochemical connection between the source and the target tissue. Furthermore, IR radiant emission can be closely tailored to reflect the incoming signal; however the potential disadvantages of INS are risks of heat-induced tissue damage by overdosed energy, and the limited depth of stimulation dependent on IR absorption properties of tissue [42].
Many researchers have found that application of continuous wave or pulsed light leads to different results in studies of wound healing and tissue regeneration [43]. A low frequency pulsed IR laser significantly stimulated bone nodule formation in rat calvarial cells in vitro with a low-energy Ga-Al-As laser (2-Hz, 830 nm, 500 mW, 0.48 3.84 J/cm 2 ) [44]. With respect to the INS, it is considered that the threshold for safety involves avoiding the heating of tissue depending on the neural targets, wavelength, pulse rates, power etc [45, 46]. INS for a cochlear implant is comparable to electrical stimulation, while other neural targets may have lower safety thresholds for INS. A pulsed diode laser with a wavelength between 1.844 1.873 μm, pulse length 35~1000 μs, repetition rate 2 Hz, was used to elicit compound action potentials. Results showed that pulse length of 35 μs was sufficient to elicit compound action potentials from the cochlea. To conduct a 50-μ compound action potential, the peak power was constant for pulse durations 100 μs~1000 μs, but showed a higher peak power at pulse length of 35 μs [47].
One possible mechanism of INS is photothermal effects caused by water absorption of energy, rather than photochemical reactions that can occur with radiation possessing greater photon energy (shorter wavelength), or photomechanical pressure waves [48]. The heat-sensitive ion channel called “transient receptor potential vanilloid 1” (TRPV1) is a possible receptor that is stimulated during INS. TRPV1 may be activated thermally by the radiant energy absorbed by water present within the neural tissue. Since most TRPV1 knockout mice had no response to IR optical stimulation of the cochlea, shown by a lack of any action potential transmitted in the auditory nerve during IR exposure (λ=1.85, 1.86 μm), this observation supported the hypothesis that TRPV1 is involved in the action potential generation through IR radiation [49]. Furthermore, isolated retinal and vestibular ganglion cells from rodents were used to observe IR laser-evoked response. By adding TRPV1 and TRPV4 channel blockers to identify the primary effectors, the study concluded that TRPV4 channels evoked the sensory neuronal response triggered by IR laser irradiation (λ=1.87μm) [50].
Intracellular Ca2+ is an important second messenger for diverse biological process, such as smooth muscle contraction, neurotransmitter release, and regulation of signaling pathways [51]. After exposure to IR radiation (1862 nm), a rapid rise in intracellular calcium was observed in the neonatal rat ventricular cardiomyocytes to that produced a pulsing frequency in the cells [52]. Using fluorescence analysis, 1862 nm IR pulses (0.2 1 Hz) could stimulate both IR-evoked and spontaneous calcium events. IR-evoked calcium events displayed a smaller amplitude and shorter time constants compared to spontaneous calcium events. A mitochondrial Ca2+ inhibitor was used and supported the hypothesis that pulsed IR radiation regulates Ca2+ in mitochondria through the mitochondrial Na+/Ca2+ exchanger and the mitochondrial Ca2+ uniporter.
In 2016, Ken Zhao et al. reviewed the applications of INS, concentrating on its ability to stimulate various types of neurons by optical radiation, including facial nerve, cochlea, vestibular system, and the cortex [53]. They concluded that IR radiation was primarily absorbed by water.”
Periodic IR femtosecond laser radiation (780 nm) was observed to synchronize single or small groups of cardiomyocytes as an “optical pacemaker” [54]. In this study, the power of the IR laser was adequately adjusted to induce periodic calcium release and avoid cytosolic calcium overproduction. The laser was applied with an average total power between 15 and 25 mW. The calcium response with synchronization in isolated cardiomyocytes (or a specific cell within a group of cardiomyocytes) depended on the average laser power on the targeted cell.
Previous studies showed that IR pulsed radiation at 1860 nm or 790~850 nm stimulated action potentials in many different types of neural cells, such as sciatic cells, auditory nerves, and cardiomyocytes [52, 55, 56]. The semicircular canal crista ampullaris of the toadfish (which functions as the balance organ of the inner ear) was sensitive to IR radiation (1862 nm) [57]. When the sensory epithelium was irradiated by various types of IR pulses, activation of the phasic inhibitory and excitatory afferent responses were observed. However there were no phase-locked afferent nerve action potentials observed with thermal stimulation to the sensory epithelium.
Furthermore, IR laser (λ = 1450 nm and 1860 nm) can transiently inhibit the propagation of action potentials in endogenous unmyelinated and myelinated axons IR laser delivered by a 200 μm optical fiber was delivered between electrical stimulation produced by a micropipette and the nerve signal recorder. The data showed that the electrical stimulation-induced action potential was blocked by IR radiation, including aplysia muscle contraction and rat sciatic nerve conduction.
In addition, an IR pulsed laser (1.86 μm) was applied to evaluate the spatial selectivity of the acutely damaged cochlea in a guinea pig. The neural response of the inferior colliculus was transformed into spatial tuning curves in order to compare the differences between acoustically-evoked responses and IR pulse-evoked responses [58]. Most of the spatial tuning curves indicated that optical stimulation could activate selective populations of neurons in the same way as acoustic stimulation; only 10% of the profiles could not be analyzed or correlated.
The main disadvantage of INS is heat deposition in the tissue, and this could represent an obstacle against development of implantable devices for applications such as artificial cochleas. Recently, a hybrid electro-optical stimulation technique has been developed to combine INS with electrical stimulation [59, 60]. The sciatic nerve of the rat hind-limb was irradiated by a pulsed diode laser (λ = 1875 nm) during electrical stimulation. Furthermore, it was observed that an increase in nerve tissue temperature caused by the optical stimulation could enhance the hybrid electro-optical stimulation response of nerves.
3.2. IR effects on Skin: Photoaging vs Photorejuvenation
In recent years, photodermatological investigation has made enormous progress in understanding the molecular mechanisms that form the basis for the good and the bad effects that human skin can undergo in response to exposure to IR radiation. Most studies used artificial light sources for IRA illumination. This makes it possible to identify the most efficient wavelength, power, and fluence to irradiate subjects than when using environmental IR radiation from the sun containing multi-wavelengths, which may cause heat-induced MMP-1 and induced photoprotection of human skin [61].
Since human skin is consistently exposed to environmental IR radiation, this energy may indirectly or directly stimulate the production of free radicals or ROS. Many researchers have found a brief burst of IR-induced ROS may be beneficial for photorejuvenation. IR radiation (8~12 μm) is used on full-thickness skin wound healing in rats, has shown an increase in the release of the growth factor and anti-inflammatory cytokine transforming growth factor-β1 (TGF-β1) which leads to activation of fibroblasts for improved healing of wounds [31]. Additionally, IR radiation (λ= 950 nm) has been used to directly stimulate fibroblast proliferation, and this resulted in an increased fibroblast proliferation in vitro [62].
The molecular mechanism of NIR radiation (λ=810 nm) to generate mitochondrial signaling in mammalian cells is proposed to be due activation of a photoacceptor called cytochrome c oxidase (CCO). Light activation of CCO stimulates the mitochondrial respiratory chain reaction to generate ROS, and results in the activation of NF-κB in embryonic fibroblasts [13, 63]. In addition PBM absorption of IR radiation by structured intracellular water, may produce additional changes in molecular vibrational energy and affect the tertiary conformation of enzymes, ion channels and other proteins. These relatively small changes in protein structure can activate signaling pathways (such as by inositol phosphates) culminating in activation of transcription factors and changes in gene expression [64, 65].
Furthermore, primary human dermal fibroblasts were analyzed by microarray analysis after IRA radiation in vitro. Microarray analysis indicated that 599 IRA-regulated genes were differentially expressed in primary human dermal fibroblasts, which were relevant to metabolic processes in the extracellular matrix, calcium homeostasis, stress signaling, and regulation of apoptosis [17]. This research also found that IRA resulted in the generation of ROS both inside and outside of the mitochondria. The authors proposed that three major signaling pathways may be involved to activate gene expression, including mitogen-activated protein kinases (MAPKs), calcium, and the interleukin 6/signal transducer and activator of transcription 3 (STAT3) pathways. Additionally, the IRA-induced genes were significantly different compared to UV-induced genes. This finding implies that different wavelengths of light may lead to specific signaling pathways in human dermal fibroblast.
However, IR-induced free radicals and ROS can be a double-edged sword, at low doses they can activate protective responses, but at high doses ROS can damage organelles and cells in the skin resulting in photoaging. Many studies have indicated that IR radiation ranging from 760~1,000 nm is involved in photoaging and photocarcinogenesis of human skin [66]. The mechanism of IR radiation damaging the skin is based on upregulation of matrix metalloproteinase-1 (MMP-1), which is mediated by stimulation of the p38-MAPK pathway and extracellular signal regulated kinase 1/2 (ERK1/2) signaling pathways in response to IRA radiation. When human skin is irradiated with single or multiple applications (once a week for 4 weeks) of IR radiation, it can lead to different expression of type I procollagen, and higher expression of TGF-β1, -β2, and -β3 [67, 68].
Additionally, an IR lamp with a maximum emission at 1100~1120 nm was used to irradiate human skin. Blood vessels stained by the endothelial cell marker CD31 were increased by IR radiation probably through up-regulation of vascular endothelial growth factor (VEGF) and the down-regulation of the antiangiogenic factor thrombospondin-2 (TSP-2) in the skin epidermis [69].
IRA radiation-induced free radicals can decrease antioxidants such as carotenoids to different degrees in human skin. Especially the carotenoid, lycopene decreased quickly compared to beta-carotene [70]. Many non-invasive measurements have been used to investigate the production of free radicals in human skin during exposure to IR radiation, such as resonance Raman spectroscopy, reflection spectroscopy, and skin color measurement [71, 72].
Electron paramagnetic resonance spectroscopy is based on the reso
nant absorption of microwave radiation by matching the energy difference of the spins of free unpaired electron in a magnetic field, and spin reversal and absorption of microwave energy can be measured [73]. The rotational effect in tissue water with a significant damping induced by the resonant absorption of microwave radiation should be considered to avoid a consequence of the high impedance at this frequency level (109Hz). In previous studies, resonance Raman spectroscopy and electron paramagnetic resonance spectroscopy were used in parallel on the skin of 17 volunteers. Nitroxide radicals (with a free unpaired electron on the nitrogen atom) were used to determine the antioxidative capacity of the skin in vivo. The results showed that the rate of decrease of nitroxide was correlated with the concentration of cutaneous carotenoids [74].
The antioxidative mechanism of carotenoids is to quench singlet oxygen by its conjugated carbon double-bond system. The concentration of carotenoids could report the entire antioxidant levels of human skin [75]. Resonance Raman spectroscopy is a non-invasive optical method to eliminate the influences of heterogeneities and measure the concentration of carotenoids in the skin [76].
Moreover, IRA-induced depletion of carotenoids in ten volunteers was analyzed by resonance Raman spectroscopy and the depth distribution of the carotenoid concentration on the volar forearm was determined by the use of confocal Raman microscopy [77]. The results showed that after exposure to IRA radiation, the carotenoid concentration was reduced immediately and lasted until 60 minutes post-exposure. The original level of the initial antioxidant concentration had recovered at 24 hours post-exposure.
ROS caused by high doses of IRA can reduce antioxidants significantly in vivo. This should be taken into account and the skin should only be exposed to low-to-moderate doses of IRA radiation to avoid tissue damage and photoaging. Barolet et al in an opinion article entitled (Infrared and skin: friend or foe?) [3] emphasized the pronounced biphasic dose effects of IR on the skin. The beneficial effects of low doses of IR on the skin included photoprotection against UV-induced damage, photorejuvenation, reduction of pigmented lesions, and fewer fine lines and wrinkles. Therefore the evidence as a whole supports the conclusion that optimal light parameters are critical for different application of LLLT and PBM especially on the skin, but on other organ systems as well [78].
IR-induced heat action can be pathological for the skin. When the skin temperature exceeds 39 C during IR irradiation, it can induce ROS generation and pathological effects through changes in structural integrity caused by enzyme induction in the skin [79]. In addition, the regulation of aquaporin 3 protein expression is involved in the functional mechanisms of intense pulsed light at 560 nm, which plays an important role in skin homeostasis to transport waster and small molecule solutes [80].
As mentioned above high skin temperatures can activate the heat-sensitive ion channels of the TRPV1 family, increasing the concentration of intracellular Ca2+ inside the cell, and subsequent activation of signaling pathways [81, 82].
3.3. Antitumor Action
Over the past decade, a number of studies have found that IR radiation can give rise to some DNA damage in cancer cells [83–85]. The proposed mechanism is related to oxidative stress. IR affects the electron transport chain to generate ROS, which does not only stimulate signal transduction at moderate levels, but can also damage cellular organelles directly when generated at excessive levels. IR-induced mitochondrial ROS were reported to be able to damage human mitochondrial DNA (mtDNA) that takes the form of a 16,559-bp circular double-stranded molecule containing 37 genes, and resulting in alteration of respiratory chain function [86]. Furthermore, mtDNA mutations play an important role in pathological abnormalities. Up to the present time more than 100 point mutations in mtDNA have been found [87].
The mutation frequency of mtDNA is significantly higher than that of nuclear DNA. This is because the DNA repair mechanisms against oxidative stress induced DNA damage are not as effective in mitochondri as they are in the nucleus of the cell. This applies to bulky DNA lesions or photoproducts, such as pyrimidine (6–4) pyrimidone photoproducts or cyclopyrimidine dimers [88]. Furthermore, mtDNA is located right next to the electron transport chain, which has the highest IR-induced ROS generation side the cell. Therefore, ROS is highly likely to cause mtDNA damage and trigger a cascade of apoptosis and cell death.
To clarify the intracellular location of IRA-induced ROS, antioxidants were used to pretreat human fibroblasts [17]. The antioxidant N-acetyl-cysteine can elevate intracellular glutathione level [89], scavenge reactive oxygen species in all the different cellular compartments, and therefore is able to inhibit all changes in expression of IRA-induced genes. However, IRA still activates ROS-related genes if MitoQ is used as an antioxidant, which was designed to scavenge ROS specifically originating inside the mitochondria [90]. This implies that other IRA-activated chromophores in different cellular compartments may be involved in IRA-induced ROS formation, and not exclusively limited to the mitochondria. Moreover, the IRA-induced expression of MMP-1 enzyme in primary human skin fibroblasts can be decreased by antioxidants, such as ascorbic acid, (α)-tocopherol, epigallocatechingallat, (−)-epicatechin, or phenylpropionic acid [91]. In addition, the enzyme MMP-1 has been proposed to behave like a “Brownian motion ratchet”, governed by water dynamics that can be stimulated by IR light. For instance, activated collagenase (MMP-1) acts as a molecular ratchet with a role in tissue remodeling and cell matrix interactions [92]. Therefore, appropriate antioxidants could be applied to protect against premature skin aging induced by IRA radiation. The human breast cancer cell lines MDA-MB-231, MCF7, T47D, and normal breast epithelial cells (184B5) were irradiated by MIR (λ= 3.0~5.0 μm). Quantitative proteomics analysis was used to study the MIR-regulated physiological responses of breast cancer cells, including G2/M cell cycle arrest, remodeling of the microtubule network to an astral pole arrangement, alteration in the actin lament cytoskeleton, and reduced cell migration activity[85].
Chang et al. demonstrated that IR radiation (3~5 μm) could cause swelling and cell cycle arrest in the G2/M phase in A549 lung cancer cells [84]. IR radiation could also inhibit the phosphorylation of cyclin-dependent kinase 1 (CDK1) and cyclin B1, resulting in the arrest of cell cycle progression. In addition, the perinuclear distribution of actin filaments in the lung cancer cells implies that oxidative stress generated by IR radiation influenced the cell cycle arrest, reorganization of the cytoskeleton and affected the balance of antioxidants [93]. This study also found that IR radiation triggered the ATM/ATR-p53-p21 axis in response to DNA damage, leading to the formation of 53BP1 and c-H2AX nuclear foci, and activation of the ATM/ATR-p53-p21 pathway involved in DNA repair. These data imply that IR radiation induced the DNA repair system in response to DNA damage.
FIR (4~1000 μm) radiation induces molecular vibrations leading to increased temperatures inside the cells, and it may cause a local heat stress environment. The induction of heat shock protein (HSP) 70 can inhibit release of cytochrome c from mitochondria which is an upstream step in apoptosis [94]. Previous literature has shown that low basal expression of HSP70 and changes in cellular morphology were observed in the FIR sensitive cell lines HSC3, Sa3 and A549 [95].
In addition, FIR induced cell hypertrophy and inhibited proliferation of A549 (lung), HSC3 (tongue) and Sa3 (gingiva) cancer cells by G2/M cell cycle arrest through the over expression of ATF3 gene [96]. The ATF3 gene is involved in responding to changes in the extracellular or intracellular micro-environments, cellular homeostasis, cell cycle, and cell death [97]. However IR radiation did not affect the expression of the ATF3 gene and cell hypertrophy in A431 (vulva) or MCF7 (breast) cancer cells. These results indicate that FIR radiation suppresses the proliferation of cancer cells depending on the specific cell type and may be an effective treatment in some kinds of cancer.
Previous research has demonstrated that ionizing radiation therapy combined with paclitaxel can increase the therapeutic effects [98]. Paclitaxel stabilizes microtubules and leads to cell death by inhibiting chromosome segregation, disturbing spindle assembly during cell division, and causing cell cycle arrest in the G2/M phase. Furthermore, paclitaxel also activates several mitochondrial cytotoxicity pathways by altering the permeability of pores in the mitochondria, dissipating mitochondrial membrane potential, releasing cytochrome c from the inter-membranous space, and forming ROS [99]. HeLa human cervical cancer cells were treated with paclitaxel combined with MIR irradiation (3.6, 4.1 and 5.0 μm) and showed improved antitumor effects [100]. IR has the potential to reduce the dosage of paclitaxel in clinical anticancer chemotherapy to avoid paclitaxel-induced severe side-effects, such as lower white blood cell counts, hair loss, diarrhea, mouth sores, and hypersensitivity reactions.
3.4. Neural and Adipose Regeneration
Transcranial brain stimulation with IR radiation is the use of coherent or non-coherent light to rehabilitate neurodegenerative brain diseases or traumatic brain injury, and modulate a neurobiological function in a non-thermal effect; however the molecular mechanism of IR brain stimulation is still unclear.
In order to clarify the cellular mechanism of NIR laser treatment on acute ischemic stroke patients, the rabbit small clot embolic stroke model was used to evaluate the cortical ATP content after 808 nm laser treatment [101]. A NIR laser in pulsed wave mode or continuous mode could elevate ATP content in the rabbit cortex compared to the sham embolized rabbits, especially pulsed wave mode gave a significantly larger increase in cortical ATP content.
An 810 nm Ga-Al-As diode laser pulsed at 10-Hz, 100-Hz and continuous mode, with a power density of 50-mW/cm2 for 12-minutes, was used to illuminate the head of mice with an experimental traumatic brain injury (TBI). Mice were sacrificed and analyzed at 2, 15 and 28 days post-TBI. As well as lesion size and quantity of ATP production, the 10 Hz pulse frequency had the best effect on neurological performance [102]. This study suggested that the 4~10 Hz rhythm occurring in the hippocampal region in the normal brain of mice, could enter into positive resonance with the 10 Hz laser pulse frequency to enhance the neurorehabilitation of TBI mice.
The 808 nm laser could also promote cerebral blood flow and increase nitric oxide levels in mice [103]. It was suggested that the IR laser could promote cerebral circulation through NO release as well as activating neuroprotective pathways to reduce the numbers of apoptotic cells in the hippocampus.
There are many hypotheses to explain the degeneration of neuronal processes in Parkinson's disease, including decreased levels of dopaminergic neurons in the substantia nigra, the presence of cytoplasmic inclusions and abnormal alpha-synuclein-positive axonal swellings in surviving neurons [104].
In an attempt to investigate the reduced axonal transport-induced in Parkinson's disease, the velocity of mitochondrial movement in human transmitochondrial cybrid neuronal cells were measured during 810 nm diode laser treatment [105]. Cybrids are neurons that have has their own mitochondria replaced with diseased mitochondria obtained from other cells (for instance those obtained from Parkinson’s disease patients). The speed of mitochondrial movement in Parkinson's disease cybrid neurites were significantly increased after exposure to IR radiation for two hours. It was proposed that IR laser treatment could inhibit neurodegenerative symptoms in patients with Parkinson's disease.
In addition, amyloid-β protein precursor transgenic mice (a mouse model of Alzheimer’s disease) were treated 3 times/week with various doses of 808nm IR laser [106]. The levels of brain amyloid-β peptide, plasma amyloid-β peptide, and cerebrospinal fluid amyloid-β peptide, and the numbers of amyloid-β plaques in the brain were all reduced by IR laser treatment in a dose-dependent manner. In addition, IR laser-induced ATP generation may also enhance neuronal preservation and inhibit amyloid plaque formation.
These data taken together indicate that IR radiation may induce promotion of cell viability and growth factors, which elicit potential therapeutic effects on the brain injury or degenerative brain disease. Brain disorders including TBI, Alzheimer’s disease, Parkinson's disease, and stroke could be benefited by IR-induced ATP synthesis, growth factor production, anti-inflammatory effects and anti-apoptosis. [107]. Furthermore, a recent study also indicates that the proliferation and differentiation of adipose-derived stem cells are regulated by 980nm IR radiation that is proposed to affect temperature-gated calcium ion channels, while 810nm IR radiation stimulated ATP production via absorption of photons by CCO [108].
It should be noted that IR 810 nm is not only absorbed by CCO, but also absorbed at low levels by water. While the 980 nm IR is not much absorbed by CCO, it is mainly absorbed by water [25].
Table 1 summarizes reports of IR radiation being used to interact with cells and tissues. It also highlights some of the medical applications of IR radiation. The wavelengths of the light sources are proposed to match the absorption spectrum of CCO or water molecules.
Table 1
Various medical applications of IR radiation for different cells and tissue tissues.
Medical AppicationAuthor, referenceTargetLight source or materialWave lengthsResultsWound Healing Toyokawa et al. [31] Skin wound in rat Ceramic-coated sheet 5.6 ~ 25 μm (maximal intensity of 8 ~ 12 μm) Promoted wound healing and expression of TGF-β1
Wound Healing Gupta et al. [109] Dermal abrasions in mice Diode laser 810 nm Enhanced collagen accumulation and healing effects
Wound Healing Santana-Blank et al. [110, 111] Soft tissues in rat Diode laser 904nm Promotes wound healing and exclusion zone (EZ) growth (1H-NMR 1/T2)
Wound Healing Santana-Blank et al. [111]
Rodríguez-Santana et al.[112] Soft tissues in rat Diode laser 904nm Promotes wound healing, membrane effect measured by 1H-NMR tau(c)
Neural Stimulation Wells et al. [55] Rat sciatic nerve Free electron laser 2.1, 3.0, 4.0, 4.5, 5.0, and 6.1 μm Generated a spatially selective response in small fascicles of the sciatic nerve
Neural Stimulation Jenkins et al. [113] Adult rabbit heart Diode laser 1.851μm Induced optical pacing of the adult rabbit heart
Neural Stimulation Izzo et al. [56] Gerbils auditory nerve Holmium:YA G laser 2.12 μm Optical radiation stimulated the cochlear response amplitudes
Neural Stimulation Duke et al. [60] Rat sciatic nerve Diode laser 1.875μm Hybrid electro-optical stimulation generated sustained muscle contractions and reduced the laser power requirements
Neural Stimulation Shapiro et al. [19] HEK-293T cells Diode laser 1.889 μm Altered the membrane electrical capacitance during optical stimulation transiently
Photoaging Darvin et al. [76] Human skin Radiator equipped with a water filter 600 ~1500 nm Formed free radicals and decreased content of β carotene antioxidants
Photoaging Schroeder et al. [91] Human dermal fibroblasts Water-filtered IR-A irradiation source 760~14 40 nm Increased expression of MMP-1 in the dermis
Antitum or Action Tsai et al. [100] HeLa cervical cancer cell Waveguide Thermal Emitter 3.6, 4.1 or 5.0 μm Caused a collapse of mitochondria l membrane potential and an increase in oxidative stress.
Antitum or Action Chang et al. [84] Breast cancer cells and normal breast epithelial cells. Blackbody source equipped with 3~5 μm filter 3~5 μm Induced G 2 /M cancer cell cycle arrest, remodeled the microtubule network and altered the actin filament formation
Antitum or Action Tanaka et al. [83] A549 lung adenocarcinoma cells NIR radiator equipped with a water filter 1.1~1.8 μm Activated the DNA damage response pathway
Antitum or Action Yamashita et al. [96] A431 (vulva), A549 (lung), HSC3 (tongue), MCF7 (breast) and Sa3 (gingiva) cancer cells FIR radiant-panel incubator by coating a carbon/silica/a luminum oxide/titanium oxide ceramic 4~20 μm (maxim um at 7 to 12 μm) Suppressed the proliferation of cancer cells through enhancing the expression of ATF3 gene
Antitum or Action Santana-Blank et al.[114] Solid tumor Clinical trial Diode laser 904nm 88% anticancer effect. Ten years follow up
Antitum or Action Santana-Blank et al.[115] Solid tumor cytomorphology Diode laser 904nm Selective apoptosis, necrosis, anoikis in tumor tissues of cancer patients
Antitum or Action Santana-Blank et al. [116] Solid tumor T2wMRI-Microde nsitometry Diode laser 904nm Evidence of interfacial water exclusion zone (EZ) as a predicator of anti-tumor response in cancer patients
Antitum or Action Santana-Blanket al.[117] Solid tumor serum levels of cytokines of peripheral leucocyte subsets Diode laser 904nm Immuno-modulation in cancer patients of TNF-α sIL-2R and CD4+CD45 RA+ and CD25+ activated
Brain Neural Regeneration Naeser et al. [118] Mild traumatic brain injury NIR diodes 870 nm Improved cognitive function, sleep and post-traumati c stress disorder symptoms
Brain Neural Regeneration Lapchak et al. [101] Strokes in embolized rabbits Laser source 808 nm Increased cortical ATP content
Adipose Regeneration Wang, Y., et al. [108] human adipose-derived stem cells Diode laser 810 nm
980nm Stimulate the proliferation and differentiatio n
4 Discussion
LLLT and/or PBM has been used for a wide range of different medical indications in recent years, and the cellular and molecular action mechanisms of LLLT are now better understood than in past decades.
Most studies have proposed that the chromophores responsible for PBM effects can be primarily classified as either mitochondrial chromophores such as CCO.
Previous studies have identified the chromophore of PBM using red or NIR wavelengths to be mitochondrial CCO. CCO is one of the four protein complexes (unit IV) composing the electron transport chain that carries out electron transport at the inner mitochondrial membrane, finally generating an electrochemical proton gradient for the final enzyme ATP synthase (unit V) to transform ADP (adenosine diphosphate) to produce ATP [119, 120]. LLLT can increase the CCO enzyme activity to faciliate electron transport and increase ATP production [121]. Furthermore, it has been found that the action spectrum for the biological response in the NIR range matches the absorption spectra of CCO in the NIR attributed to mitochondrial chromophores [63, 122–124]. The absorbance of cytochrome c oxidase in the visible and NIR spectral regions show a good match to the action spectrum for the increase of DNA synthesis in mammalian cells. CCO has two copper centers, CuA and CuB and two heme centers, hemeA and hemeB. Each of these metal centers can be in an oxidized or a reduced state giving a total of 16 possibilities. Different photoacceptors have been attributed to various redox states of CCO, the 820 nm band has been attributed to the oxidized form of CuA chromophore of CCO, the 760 nm band to the reduced foem of CuB, the 680 nm band to the oxidized CuB , and the 620 nm band to the reduced CuA [13, 63].
On the other hand, several other studies have indicated that another possible mechanism of PBM especially at FIR and MIR wavelengths is absorption of radiation by water molecules. Pollack et al. demonstrated that radiant energy can generate an exclusion zone (EZ) in a water interface that possesses the correct type of hydrophilic/hydrophobic balance [65, 125]. EZ water can store electrical charges, and can release up to 70% of the input energy.
Cellular membranes are characterized by the presence of a thin (nanometer) layer of water that builds up on hydrophobic surfaces [126]. Very low amounts of non-heating IR radiation can deliver relatively small amounts of vibrational energy to the nanostructured water layers and may be able to perturb its structure and that of neighboring molecules without causing any bulk heating effect (i.e. without causing any measurable rise in temperature) [127]. Intramitochondrial water viscosity gradients have been identified by the technique of nano-indentation [128]. ATP synthesis can be decreased and increased in response to modulation of reactive oxygen species levels caused by non-thermal levels of NIR. The possible control mechanism of this “mitochondrial nanomotor” is that the NIR could increase ATP turnover by reducing the viscosity of the interfacial water layers. Recently, Santana-Blank et al. proposed that external electromagnetic (light) energy could activate oxygen-dependent and oxygen-independent pathways based on water light interactions [129]. As a result of water light interactions and energy transfer mechanisms, IR generates interfacial EZ-water as a selective rechargeable electrolytic bio-battery [130]. Light energy in oxygen-dependent pathways generates high-energy molecules called nucleotide-phosphates including ATP and GTP. Water light interactions in the oxygen-independent pathway, lead to photoinduced non-linear oscillations in water that could provide energy for cellular reactions, including metabolism, signaling, and gene transcription.
Recently Wang et al showed [108] that two different NIR wavelengths affected adipose-derived stem cells by distinctly different mechanisms of action. 810-nm laser was proposed to activate CCO leading to ATP production and a brief burst of ROS, but had no effect on intracellular calcium. By contrast 980 nm laser also increased ATP and ROS, but at much lower fluences (one tenth to one hundredth), and increased cytosolic calcium while at the same time decreasing mitochondrial calcium. The actions of 980 nm NIR but not the actions of 810 nm NIR could be abrogated by inhibitors of calcium ion channels such as TRPV. Heating up the cells or cooling down the cells abrogated the effects of 980 nm but not 810 nm. This study suggested that 980 nm could work by affecting nanostructured water layers in TRPV ion channels while 810 could directly activate CCO enzyme activity. Figure 2 graphically summariuzes the two most important proposed biological mechanisms of action of IR.
Proposed mechanisms of action of IR at a molecular and cellular level. TRPV = transient receptor potential vanilloid; ROS = reactive oxygen species; ATP = adenosine triphosphate.
In addition to understanding the photobiological mechanisms of LLLT/PBM using FIR/MIR and NIR wavelengths, it is important to design the light parameters with regard to clinical experience and the desired therapeutic goal for the optimum medical and biological effects as shown in Figure 3. In clinical practice, the biphasic dose response effect is crucially important for optimal clinical results [30]. Another guiding principle is that repeating the treatment daily (or even more or less often) until the wound is healed or the disease remission is observed is better than only one single application of LLLT. LLLT can be compared to a nutrient food for the human body; an adequate daily intake is best.
Overview of determinants and factors to be considered in IR therapy
All matter is ultimately composed of charged particles such as sub-atomic particles, electrons, protons etc. When electromagnetic radiation impinges on matter, the charged particles will absorb energy leading to oscillations depending on the energy of the individual photons (wavelength). Visible light is generally absorbed by electrons in molecular orbitals, while IR energy is generally absorbed by bonds within molecules leading to increased vibrational modes such as twisting, stretching, and bending. Both kinds of energy may transform and dissipate into other molecular vibrations in the form of increased thermal energy (temperature).
How are we to distinguish between NIR and FIR absorption that interact with different elements the tissue structure (water, proteins, amino acids, lipids, etc.). It’s an interesting question, because we cannot assume that the optical characteristics of the radiation remain the same, because NIR and FIR could be absorbed and re-radiated as different electromagnetic wavelengths by the tissue chromophores within a very short period of time. It is possible that the final photobiological result originates from a variety of sources, including the original incident light photon absorption, the different re-radiated electromagnetic waves arising from cellular structural molecules, and induction of electromagnetic fields that affect energy metabolism within the cells.
Tissue optics describes mathematical modeling approaches to analyze how photons of different wavelengths interact with tissue. Photons can either be absorbed or scattered (either inelastically or elastically). On the macroscopic scale, the Monte Carlo simulation tool has been applied for studying the light penetration and absorption in human skin during LLLT. Nasouri et al. simulated laser propagation through a three-layer human skin model in the spectral range from 1000 to 1900 nm [131]. This type of analysis is necessary to design parameters to maximze the depth of light penetration into tissue, without any risk of causing thermal damage to the upper layers of the skin. Additionally, the beam profile of the laser spot, which can be uniform or Gaussian can increase the local volumetric dosage, and is important when selecting wavelength and laser power in LLLT.
Overall the mechanisms of action of IR radiation can be divided into two broad groups listed in Table 2. More studies are clearly needed to investigate the mechanisms of IR radiation in the medical and biochemical arenas.
Table 2
Various aspects on the mechanisms of IR radiation
Energy Transfer mechanismSignal Pathway Mechanism
Electrical capacitance of cells regulated by IR
Cellular structures (water, proteins, amino acids, lipids, etc.)
Exclusion zone generated in water acts as a re-chargeable biological battery
Interaction between IR and water molecules
IR is absorbed and re-radiated as different electromagnetic wavelengths by tissue chromophores.
IR affects cellular redox state in mitochondria and modulates reactive oxygen species and ATP production.
Stimulation of nitric oxide, cytochrome c oxidase, transcription factors, cytokines, growth factors, and inflammatory mediators, etc.
Signaling via light or heat sensitive ion channels (Ionic pumps and molecular motors)[132]
Signaling via cyclic AMP/GMP and G-protein coupled receptors, and inositol phosphate [132]
IR induces in bulk water the release and transport of protons, activating membrane signaling pathways and transmembrane ion channel effects [133].
5 Conclusion
IR appears to be more versatile than other electromagnetic wavelengths (such as visible light) as it can induce neural stimulation effects as well as promoting a wide range of therapeutic benefits in cells or tissues. An increasing number of new reports in recent years have indicated that different forms IR application have clear clinical benefits, and the mechanisms of IR are becoming clarified. Moreover therapeutic levels of IR can be delivered using devices without any external power source, simply by using the heat production of the human body to drive emission of FIR from materials containing minerals.
In this review, we have summarized the up-to-date literature reports about medical uses of IR radiation, including neural stimulation (direct activation of neural tissue by IR radiation), photoaging (emerging evidence that IR can have biphasic effects on the skin), antitumor action (IR can inhibit cancer cell proliferation and potentiate the therapeutic effectiveness of chemotherapy), and brain neuroprotection (novel neuroprotective treatments for stroke, TBI and neurodegenerative disorders (IR radiation therapy for Alzheimer's and Parkinson's diseases [134]). Clinical evidence has demonstrated that IR can selectively induce cell death by apoptosis, necrosis and anoikis. In parallel, it induces cell differentiation as a physiological response opposed to proliferation [135, 136].
Studies on dressings or clothing made out of IR-emitting materials, have shown they can elevate blood circulation and relieve fatigue of soccer players. Therefore this mode of application could be a more flexible method for portable, lifestyle-enhancing applications (e.g., outdoor activities, sleeping, home-care) and for treating medical conditions compared with traditional IR light sources and saunas.
Highlights
Infrared is electromagnetic radiation with a wavelength 750–100,00 nm.
Short IR is proposed to be absorbed by cytochrome c oxidase in mitochondria
Long IR is proposed to be absorbed by water in heat-gated ion channels
Neural stimulation can be carried out by focused IR laser spots
IR therapy can be carried out by IR light sources or IR emitting materials
Cancer therapy and neuroprotection are effective applications.
Acknowledgments
MR Hamblin was supported by US NIH grants R01AI050875 and R21AI121700
ABBREVIATIONS
ATP adenosine triphosphate
CCO cytochrome c oxidase
CDK cyclin-dependent kinase
ERK extracellular signal-regulated kinase
EZ exclusion zone
INS infrared neural stimulation
MAPKs mitogen-activated protein kinases
MMP matrix metalloproteinase
ROS reactive oxygen species
TGF transforming growth factor
TRPV transient receptor potential vanilloid
TSP thrombospondin
VEGF vascular endothelial growth factor
Footnotes
Publisher's Disclaimer: This is a PDF file of an unedited manuscript that has been accepted for publication. As a service to our customers we are providing this early version of the manuscript. The manuscript will undergo copyediting, typesetting, and review of the resulting proof before it is published in its final citable form. Please note that during the production process errors may be discovered which could affect the content, and all legal disclaimers that apply to the journal pertain.
References
1. Vatansever F, Hamblin MR. Far infrared radiation (FIR): its biological effects and medical applications. Photonics Lasers Med. 2012;4:255–266. [PMC free article] [PubMed] [Google Scholar]
2. Tsai SR, et al. Low-level light therapy potentiates NPe6-mediated photodynamic therapy in a human osteosarcoma cell line via increased ATP. Photodiagnosis Photodyn Ther. 2015;12(1):123–30. [PMC free article] [PubMed] [Google Scholar]
3. Barolet D, Christiaens F, Hamblin MR. Infrared and skin: Friend or foe. J Photochem Photobiol B. 2015;155:78–85. [PMC free article] [PubMed] [Google Scholar]
4. Oosterveld FG, et al. Infrared sauna in patients with rheumatoid arthritis and ankylosing spondylitis. A pilot study showing good tolerance, short-term improvement of pain and stiffness, and a trend towards long-term beneficial effects. Clin Rheumatol. 2009;28(1):29–34. [PubMed] [Google Scholar]
5. Tuby H, Maltz L, Oron U. Low-level laser irradiation (LLLI) promotes proliferation of mesenchymal and cardiac stem cells in culture. Lasers Surg Med. 2007;39(4):373–8. [PubMed] [Google Scholar]
6. AlGhamdi KM, Kumar A, Moussa NA. Low-level laser therapy: a useful technique for enhancing the proliferation of various cultured cells. Lasers in medical science. 2012;27(1):237–249. [PubMed] [Google Scholar]
7. Schiffer F, et al. Psychological benefits 2 and 4 weeks after a single treatment with near infrared light to the forehead: a pilot study of 10 patients with major depression and anxiety. Behavioral and Brain Functions. 2009;5(1):46. [PMC free article] [PubMed] [Google Scholar]
8. Rojas JC, Gonzalez-Lima F. Low-level light therapy of the eye and brain. Eye Brain. 2011;3:49–67. [PMC free article] [PubMed] [Google Scholar]
9. Johnstone DM, et al. Turning On Lights to Stop Neurodegeneration: The Potential of Near Infrared Light Therapy in Alzheimer's and Parkinson's Disease. Frontiers in Neuroscience. 2016;9(500) [PMC free article] [PubMed] [Google Scholar]
10. Anders JJ, Lanzafame RJ, Arany PR. Low-level light/laser therapy versus photobiomodulation therapy. Photomed Laser Surg. 2015;33(4):183–4. [PMC free article] [PubMed] [Google Scholar]
11. Passarella S, Karu T. Absorption of monochromatic and narrow band radiation in the visible and near IR by both mitochondrial and non-mitochondrial photoacceptors results in photobiomodulation. Journal of Photochemistry and Photobiology B: Biology. 2014;140:344–358. [PubMed] [Google Scholar]
12. Bashkatov AN, et al. Optical properties of human skin, subcutaneous and mucous tissues in the wavelength range from 400 to 2000 nm. Journal of Physics D: Applied Physics. 2005;38(15):2543. [Google Scholar]
13. Karu TI. Mitochondrial Signaling in Mammalian Cells Activated by Red and Near-IR Radiation. Photochemistry and Photobiology. 2008;84(5):1091–1099. [PubMed] [Google Scholar]
14. De Freitas LF, Hamblin MR. Proposed Mechanisms of Photobiomodulation or Low-Level Light Therapy. IEEE Journal of Selected Topics in Quantum Electronics. 2016;22(3):7000417. [PMC free article] [PubMed] [Google Scholar]
15. Irvine RF, Schell MJ. Back in the water: the return of the inositol phosphates. Nat Rev Mol Cell Biol. 2001;2(5):327–338. [PubMed] [Google Scholar]
16. Santana-Blank LA, Rodríguez-Santana E, Santana-Rodríguez KE. Photo-infrared pulsed bio-modulation (PIPBM): a novel mechanism for the enhancement of physiologically reparative responses. Photomedicine and Laser Therapy. 2005;23(4):416–424. [PubMed] [Google Scholar]
17. Calles C, et al. Infrared A radiation influences the skin fibroblast transcriptome: mechanisms and consequences. J Invest Dermatol. 2010;130(6):1524–36. [PubMed] [Google Scholar]
18. Rojas JC, Gonzalez-Lima F. Neurological and psychological applications of transcranial lasers and LEDs. Biochemical Pharmacology. 2013;86(4):447–457. [PubMed] [Google Scholar]
19. Shapiro MG, et al. Infrared light excites cells by changing their electrical capacitance. Nat Commun. 2012;3:736. [PMC free article] [PubMed] [Google Scholar]
20. Hamblin MR, de Sousa MVP, Agrawal T. Handbook of Low-Level Laser Therapy. Pan Stanford Publishing Pte. Ltd; 2016. [Google Scholar]
21. Pollack GH, Cameron IL, Wheatley DN. Water and the Cell. Springer; 2006. [Google Scholar]
22. Yao J, Liu B, Qin F. Rapid temperature jump by infrared diode laser irradiation for patch-clamp studies. Biophys J. 2009;96(9):3611–9. [PMC free article] [PubMed] [Google Scholar]
23. Szigeti GP, Hegyi G, Szasz O. Conference Papers in Medicine. Hindawi Publishing Corporation; 2013. Hyperthermia versus Oncothermia: Cellular Effects in Cancer Therapy. [Google Scholar]
24. McLaughlin S. The Electrostatic Properties of Membranes. Annual Review of Biophysics and Biophysical Chemistry. 1989;18(1):113–136. [PubMed] [Google Scholar]
25. Hale GM, Querry MR. Optical Constants of Water in the 200-nm to 200- μm Wavelength Region. Applied Optics. 1973;12(3):555–563. [PubMed] [Google Scholar]
26. Gkogkos AS, et al. Effect of Nd: YAG low level laser therapy on human gingival fibroblasts. International journal of dentistry. 2015 [PMC free article] [PubMed] [Google Scholar]
27. Hamblin MR, et al. SPIE BiOS. International Society for Optics and Photonics; 2015. Low level laser (light) therapy and photobiomodulation: the path forward. [Google Scholar]
28. Santana-Blank LA, et al. Phase I trial of an infrared pulsed laser device in patients with advanced neoplasias. Clinical cancer research. 2002;8(10):3082–3091. [PubMed] [Google Scholar]
29. Rodriguez-Santana E, et al. Photo-infrared pulsed biomodulation in age-related macular degeneration associated to neurological disease: one interventional case report and mini-review. J Chinese Clin Med. 2008;3:470–477. [Google Scholar]
30. Huang Y-Y, et al. Biphasic dose response in low level light therapy an update. Dose-Response. 2011;9(4) dose-response. 11–009. Hamblin. [PMC free article] [PubMed] [Google Scholar]
31. Toyokawa H, et al. Promotive effects of far-infrared ray on full-thickness skin wound healing in rats. Exp Biol Med (Maywood) 2003;228(6):724–9. [PubMed] [Google Scholar]
32. Chung J, Lee S. Development of nanofibrous membranes with far-infrared radiation and their antimicrobial properties. Fibers and Polymers. 2014;15(6):1153–1159. [Google Scholar]
33. Loturco I, et al. Effects of far infrared rays emitting clothing on recovery after an intense plyometric exercise bout applied to elite soccer players: a randomized double-blind placebo-controlled trial. Biol Sport. 2016;33(3):277–283. [PMC free article] [PubMed] [Google Scholar]
34. Bagnato G, et al. Far infrared emitting plaster in knee osteoarthritis: a single blinded, randomised clinical trial. Reumatismo. 2012;64(6):388–394. [PubMed] [Google Scholar]
35. Leung TK. In vitro and in vivo studies of the biological effects of bioceramic (a material of emitting high performance far-infrared ray) irradiation. Chinese Journal of Physiology. 2015;58(3):147–155. [PubMed] [Google Scholar]
36. Leung TK, et al. Bone and joint protection ability of ceramic material with biological effects. Chin J Physiol. 2012;55(1):47–54. [PubMed] [Google Scholar]
37. Beever R. Far-infrared saunas for treatment of cardiovascular risk factors Summary of published evidence. Canadian family physician. 2009;55(7):691–696. [PMC free article] [PubMed] [Google Scholar]
38. Kihara T, et al. Repeated sauna treatment improves vascular endothelial and cardiac function in patients with chronic heart failure. Journal of the American College of Cardiology. 2002;39(5):754–759. [PubMed] [Google Scholar]
39. Tei C, et al. Waon Therapy for Managing Chronic Heart Failure Results From a Multicenter Prospective Randomized WAON-CHF Study. Circulation Journal. 2016 [PubMed] [Google Scholar]
40. Kikuchi H, et al. Effect of repeated Waon therapy on exercise tolerance and pulmonary function in patients with chronic obstructive pulmonary disease: a pilot controlled clinical trial. International journal of chronic obstructive pulmonary disease. 2014;9:9. [PMC free article] [PubMed] [Google Scholar]
41. Ikeda Y, et al. Repeated sauna therapy increases arterial endothelial nitric oxide synthase expression and nitric oxide production in cardiomyopathic hamsters. Circulation Journal. 2005;69(6):722–729. [PubMed] [Google Scholar]
42. Thompson AC, et al. Modeling of light absorption in tissue during infrared neural stimulation. J Biomed Opt. 2012;17(7):075002. [PubMed] [Google Scholar]
43. Hashmi JT, et al. Effect of pulsing in low-level light therapy. Lasers in surgery and medicine. 2010;42(6):450–466. [PMC free article] [PubMed] [Google Scholar]
44. Ueda Y, Shimizu N. Effects of pulse frequency of low-level laser therapy (LLLT) on bone nodule formation in rat calvarial cells. Journal of clinical laser medicine & surgery. 2003;21(5):271–277. [PubMed] [Google Scholar]
45. Thompson AC, Stoddart PR, Jansen ED. Optical stimulation of neurons. Current molecular imaging. 2014;3(2):162–177. [PMC free article] [PubMed] [Google Scholar]
46. Wells JD, et al. Optically mediated nerve stimulation: Identification of injury thresholds. Lasers in surgery and medicine. 2007;39(6):513–526. [PubMed] [Google Scholar]
47. Izzo AD, et al. Optical parameter variability in laser nerve stimulation: a study of pulse duration, repetition rate, and wavelength. IEEE Trans Biomed Eng. 2007;54(6 Pt 1):1108–14. [PMC free article] [PubMed] [Google Scholar]
48. Wells J, et al. Biophysical Mechanisms of Transient Optical Stimulation of Peripheral Nerve. Biophysical Journal. 2007;93(7):2567–2580. [PMC free article] [PubMed] [Google Scholar]
49. Suh E, et al. SPIE BiOS: Biomedical Optics. International Society for Optics and Photonics; 2009. Optical stimulation in mice lacking the TRPV1 channel. [Google Scholar]
50. Albert ES, et al. TRPV4 channels mediate the infrared laser-evoked response in sensory neurons. Journal of Neurophysiology. 2012;107(12):3227–3234. [PubMed] [Google Scholar]
51. McDonald A, et al. Light-induced Ca(2+) transients observed in widefield epi-fluorescence microscopy of excitable cells. Biomed Opt Express. 2012;3(6):1266–73. [PMC free article] [PubMed] [Google Scholar]
52. Dittami GM, et al. Intracellular calcium transients evoked by pulsed infrared radiation in neonatal cardiomyocytes. J Physiol. 2011;589(Pt 6):1295–306. [PMC free article] [PubMed] [Google Scholar]
53. Zhao K, et al. Stimulation of Neurons with Infrared Radiation. In: Wong BJF, Ilgner J, editors. Biomedical Optics in Otorhinolaryngology: Head and Neck Surgery. Springer New York; New York, NY: 2016. pp. 253–284. [Google Scholar]
54. Smith NI, et al. A femtosecond laser pacemaker for heart muscle cells. Opt Express. 2008;16(12):8604–16. [PubMed] [Google Scholar]
55. Wells J, et al. Application of infrared light for in vivo neural stimulation. Journal of Biomedical Optics. 2005;10(6):064003–064003. [PubMed] [Google Scholar]
56. Izzo AD, et al. Laser stimulation of the auditory nerve. Lasers Surg Med. 2006;38(8):745–53. [PubMed] [Google Scholar]
57. Rajguru SM, et al. Infrared photostimulation of the crista ampullaris. J Physiol. 2011;589(Pt 6):1283–94. [PMC free article] [PubMed] [Google Scholar]
58. Richter CP, et al. Spread of cochlear excitation during stimulation with pulsed infrared radiation: inferior colliculus measurements. J Neural Eng. 2011;8(5):056006. [PMC free article] [PubMed] [Google Scholar]
59. Duke AR, et al. Combined optical and electrical stimulation of neural tissue in vivo. Journal of Biomedical Optics. 2009;14(6):060501-060501–3. [PMC free article] [PubMed] [Google Scholar]
60. Duke AR, et al. Hybrid electro-optical stimulation of the rat sciatic nerve induces force generation in the plantarflexor muscles. Journal of neural engineering. 2012;9(6):066006–066006. [PMC free article] [PubMed] [Google Scholar]
61. Grether-Beck S, et al. Photoprotection of human skin beyond ultraviolet radiation. Photodermatology, photoimmunology & photomedicine. 2014;30(2–3):167–174. [PubMed] [Google Scholar]
62. Vinck ME, et al. Increased fibroblast proliferation induced by light emitting diode and low power laser irradiation. Lasers in Medical Science. 2003;18(2):95–99. [PubMed] [Google Scholar]
63. Karu TI, et al. Absorption measurements of a cell monolayer relevant to phototherapy: Reduction of cytochrome c oxidase under near IR radiation. Journal of Photochemistry and Photobiology B: Biology. 2005;81(2):98–106. [PubMed] [Google Scholar]
64. Kim HP. Lightening up light therapy: activation of retrograde signaling pathway by photobiomodulation. Biomolecules & therapeutics. 2014;22(6):491–496. [PMC free article] [PubMed] [Google Scholar]
65. Pollack GH. Cell electrical properties: reconsidering the origin of the electrical potential. Cell biology international. 2015;39(3):237–242. [PubMed] [Google Scholar]
66. Schieke SM, Schroeder P, Krutmann J. Cutaneous effects of infrared radiation: from clinical observations to molecular response mechanisms. Photodermatology, Photoimmunology & Photomedicine. 2003;19(5):228–234. [PubMed] [Google Scholar]
67. Soyun C, et al. Effects of Infrared Radiation and Heat on Human Skin Aging in vivo. Journal of Investigative Dermatology Symposium Proceedings. 2009;14(1):15–19. [PubMed] [Google Scholar]
68. Kim MS, et al. Regulation of type I procollagen and MMP-1 expression after single or repeated exposure to infrared radiation in human skin. Mech Ageing Dev. 2006;127(12):875–82. [PubMed] [Google Scholar]
69. Kim MS, et al. Infrared exposure induces an angiogenic switch in human skin that is partially mediated by heat. Br J Dermatol. 2006;155(6):1131–8. [PubMed] [Google Scholar]
70. Darvin ME, et al. In vivo Raman spectroscopic analysis of the influence of IR radiation on the carotenoid antioxidant substances beta-carotene and lycopene in the human skin. Formation of free radicals. Laser Physics Letters. 2007;4(4):318–321. [Google Scholar]
71. Darvin ME, et al. Formation of Free Radicals in Human Skin during Irradiation with Infrared Light. J Invest Dermatol. 2009;130(2):629–631. [PubMed] [Google Scholar]
72. Darvin ME, et al. Optical methods for noninvasive determination of carotenoids in human and animal skin. Journal of Biomedical Optics. 2013;18(6):061230–061230. [PubMed] [Google Scholar]
73. Rhodes CJ. Electron spin resonance. Part one: a diagnostic method in the biomedical sciences. Sci Prog. 2011;94(Pt 1):16–96. [PubMed] [Google Scholar]
74. Haag SF, et al. Determination of the antioxidative capacity of the skin in vivo using resonance Raman and electron paramagnetic resonance spectroscopy. Experimental Dermatology. 2011;20(6):483–487. [PubMed] [Google Scholar]
75. Foote CS, Chang YC, Denny RW. Chemistry of singlet oxygen. X. Carotenoid quenching parallels biological protection. Journal of the American Chemical Society. 1970;92(17):5216–5218. [PubMed] [Google Scholar]
76. Darvin ME, et al. Non-invasive in vivo detection of the carotenoid antioxidant substance lycopene in the human skin using the resonance Raman spectroscopy. Laser Physics Letters. 2006;3(9):460–463. [Google Scholar]
77. Fluhr JW, et al. Kinetics of carotenoid distribution in human skin in vivo after exogenous stress: disinfectant and wIRA-induced carotenoid depletion recovers from outside to inside. J Biomed Opt. 2011;16(3):035002. [PubMed] [Google Scholar]
78. Huang YY, et al. Biphasic dose response in low level light therapy. Dose Response. 2009;7(4):358–83. [PMC free article] [PubMed] [Google Scholar]
79. Darvin ME, et al. Influence of IR radiation on the carotenoid content in human skin. Optics and Spectroscopy. 2009;107(6):917–920. [Google Scholar]
80. Wu CJ, et al. Effect of intense pulsed light on the expression of aquaporin 3 in rat skin. Lasers in Medical Science. 2015;30(7):1959–1965. [PubMed] [Google Scholar]
81. Clapham DE. TRP channels as cellular sensors. Nature. 2003;426(6966):517–524. [PubMed] [Google Scholar]
82. Li WH, et al. Transient Receptor Potential Vanilloid-1 Mediates Heat-Shock-Induced Matrix Metalloproteinase-1 Expression in Human Epidermal Keratinocytes. Journal of Investigative Dermatology. 2007;127(10):2328–2335. [PubMed] [Google Scholar]
83. Tanaka Y, et al. Non-thermal DNA damage of cancer cells using near-infrared irradiation. Cancer Science. 2012;103(8):1467–1473. [PubMed] [Google Scholar]
84. Chang HY, et al. Middle infrared radiation induces g(2)/m cell cycle arrest in a549 lung cancer cells. PLoS One. 2013;8(1):e54117. [PMC free article] [PubMed] [Google Scholar]
85. Chang HY, et al. Quantitative Proteomics Reveals Middle Infrared Radiation-Interfered Networks in Breast Cancer Cells. Journal of Proteome Research. 2015;14(2):1250–1262. [PubMed] [Google Scholar]
86. Birch-Machin MA. The role of mitochondria in ageing and carcinogenesis. Clinical and Experimental Dermatology. 2006;31(4):548–552. [PubMed] [Google Scholar]
87. McKenzie M, Liolitsa D, Hanna M. Mitochondrial Disease: Mutations and Mechanisms. Neurochemical Research. 2004;29(3):589–600. [PubMed] [Google Scholar]
88. Larsen NB, Rasmussen M, Rasmussen LJ. Nuclear and mitochondrial DNA repair: similar pathways? Mitochondrion. 2005;5(2):89–108. [PubMed] [Google Scholar]
89. Kerksick C, Willoughby D. The antioxidant role of glutathione and N-acetyl-cysteine supplements and exercise-induced oxidative stress. J Int Soc Sports Nutr. 2005;2(2):38–44. [PMC free article] [PubMed] [Google Scholar]
90. Smith RAJ, Murphy MP. Animal and human studies with the mitochondria-targeted antioxidant MitoQ. Annals of the New York Academy of Sciences. 2010;1201(1):96–103. [PubMed] [Google Scholar]
91. Schroeder P, et al. Infrared radiation-induced matrix metalloproteinase in human skin: implications for protection. J Invest Dermatol. 2008;128(10):2491–7. [PubMed] [Google Scholar]
92. Saffarian S, et al. Interstitial collagenase is a Brownian ratchet driven by proteolysis of collagen. Science. 2004;306(5693):108–11. [PubMed] [Google Scholar]
93. Lee CF, et al. Oxidative stress-induced depolymerization of microtubules and alteration of mitochondrial mass in human cells. Ann N Y Acad Sci. 2005;1042:246–54. [PubMed] [Google Scholar]
94. Li CY, et al. Heat shock protein 70 inhibits apoptosis downstream of cytochrome c release and upstream of caspase-3 activation. J Biol Chem. 2000;275(33):25665–71. [PubMed] [Google Scholar]
95. Ishibashi J, et al. The effects inhibiting the proliferation of cancer cells by far-infrared radiation (FIR) are controlled by the basal expression level of heat shock protein (HSP) 70A. Med Oncol. 2008;25(2):229–37. [PMC free article] [PubMed] [Google Scholar]
96. Yamashita K. Far Infrared Ray Radiation Inhibits the Proliferation of A549, HSC3 and Sa3 Cancer Cells through Enhancing the Expression of ATF3 Gene. Journal of Electromagnetic Analysis and Applications. 2010;02(06):382–394. [Google Scholar]
97. Lu D, Wolfgang CD, Hai T. Activating Transcription Factor 3, a Stress-inducible Gene, Suppresses Ras-stimulated Tumorigenesis. Journal of Biological Chemistry. 2006;281(15):10473–10481. [PubMed] [Google Scholar]
98. Choy H, et al. Investigation of taxol as a potential radiation sensitizer. Cancer. 1993;71(11):3774–8. [PubMed] [Google Scholar]
99. Varbiro G, et al. Direct effect of Taxol on free radical formation and mitochondrial permeability transition. Free Radical Biology and Medicine. 2001;31(4):548–558. [PubMed] [Google Scholar]
100. Tsai SR, et al. The effects of narrow-band middle infrared radiation in enhancing the antitumor activity of paclitaxel. Electromagn Biol Med. 2016;35(2):106–14. [PubMed] [Google Scholar]
101. Lapchak PA, De Taboada L. Transcranial near infrared laser treatment (NILT) increases cortical adenosine-5'-triphosphate (ATP) content following embolic strokes in rabbits. Brain Res. 2010;1306:100–5. [PubMed] [Google Scholar]
102. Ando T, et al. Comparison of therapeutic effects between pulsed and continuous wave 810-nm wavelength laser irradiation for traumatic brain injury in mice. PLoS One. 2011;6(10):e26212. [PMC free article] [PubMed] [Google Scholar]
103. Uozumi Y, et al. Targeted increase in cerebral blood flow by transcranial near-infrared laser irradiation. Lasers Surg Med. 2010;42(6):566–76. [PubMed] [Google Scholar]
104. Foltynie T, Brayne C, Barker RA. The heterogeneity of idiopathic Parkinson's disease. J Neurol. 2002;249(2):138–45. [PubMed] [Google Scholar]
105. Trimmer PA, et al. Reduced axonal transport in Parkinson's disease cybrid neurites is restored by light therapy. Mol Neurodegener. 2009;4:26. [PMC free article] [PubMed] [Google Scholar]
106. De Taboada L, et al. Transcranial laser therapy attenuates amyloid-beta peptide neuropathology in amyloid-beta protein precursor transgenic mice. J Alzheimers Dis. 2011;23(3):521–35. [PubMed] [Google Scholar]
107. Hamblin MR. Shining light on the head: Photobiomodulation for brain disorders. BBA Clin. 2016;6:113–124. [PMC free article] [PubMed] [Google Scholar]
108. Wang Y, et al. Photobiomodulation of human adipose-derived stem cells using 810nm and 980nm lasers operates via different mechanisms of action. Biochim Biophys Acta. 2016 [PMC free article] [PubMed] [Google Scholar]
109. Gupta A, Dai T, Hamblin MR. Effect of red and near-infrared wavelengths on low-level laser (light) therapy-induced healing of partial-thickness dermal abrasion in mice. Lasers Med Sci. 2014;29(1):257–65. [PMC free article] [PubMed] [Google Scholar]
110. Santana-Blank LA, et al. Short-Term bioeffects of an infrared pulsed laser device on burned rat skin monitored by transverse relaxation times (NMR) Lasers in surgery and medicine. 2000;27(5):411–419. [PubMed] [Google Scholar]
111. Santana-Blank L, et al. Water-light interaction: A novel pathway for multi hallmark therapy in cancer. International Journal of Cancer Therapy and Oncology. 2013;2(1) [Google Scholar]
112. Rodriguez-Santana E, et al. H-NMR spin-lattice and correlation times of burned soft-tissue after treatment with an infrared pulsed laser device. Lasers in surgery and medicine. 2003;33(3):190–198. [PubMed] [Google Scholar]
113. Jenkins MW, et al. Optical pacing of the adult rabbit heart. Biomed Opt Express. 2013;4(9):1626–35. [PMC free article] [PubMed] [Google Scholar]
114. Santana-Blank LA, et al. Phase I trial of an infrared pulsed laser device in patients with advanced neoplasias. Clinical cancer research. 2002;8(10):3082–3091. [PubMed] [Google Scholar]
115. Santana-Blank LA, et al. Photo-induced cytomorphologic changes in an advanced cancer phase I clinical trial. Lasers in Surgery and Medicine. 2002;30(1):18–25. [PubMed] [Google Scholar]
116. Santana-Blank L, Rodríguez-Santana E, Santana-Rodríguez KE. Photobiomodulation of aqueous interfaces: finding evidence to support the exclusion zone in experimental and clinical studies. Photomedicine and laser surgery. 2013;31(9):461–462. [PubMed] [Google Scholar]
117. SANTANA-BLANK L, et al. Evaluation of serum levels of tumour necrosis factor-alpha (TNF-α) and soluble IL-2 receptor (sIL-2R) and CD4, CD8 and natural killer (NK) populations during infrared pulsed laser device (IPLD) treatment. Clinical & Experimental Immunology. 1992;90(1):43–48. [PMC free article] [PubMed] [Google Scholar]
118. Naeser MA, et al. Significant improvements on cognitive performance post- transcranial, red/near-infrared LED treatments in chronic, mild TBI: Open-protocol study. J Neurotrauma. 2014 [PMC free article] [PubMed] [Google Scholar]
119. Chung H, et al. The nuts and bolts of low-level laser (light) therapy. Ann Biomed Eng. 2012;40(2):516–33. [PMC free article] [PubMed] [Google Scholar]
120. Capaldi RA, Malatesta F, Darley-Usmar VM. Structure of cytochrome c oxidase. Biochim Biophys Acta. 1983;726(2):135–48. [PubMed] [Google Scholar]
121. Karu T, Pyatibrat L, Kalendo G. Irradiation with He-Ne laser increases ATP level in cells cultivated in vitro. J Photochem Photobiol B. 1995;27(3):219–23. [PubMed] [Google Scholar]
122. Karu T. Photobiological fundamentals of low-power laser therapy. IEEE Journal of Quantum Electronics. 1987;23(10):1703–1717. [Google Scholar]
123. Karu TI, Kolyakov SF. Exact action spectra for cellular responses relevant to phototherapy. Photomed Laser Surg. 2005;23(4):355–61. [PubMed] [Google Scholar]
124. Karu TI. Multiple roles of cytochrome c oxidase in mammalian cells under action of red and IR-A radiation. IUBMB Life. 2010;62(8):607–10. [PubMed] [Google Scholar]
125. Musumeci F, Pollack GH. High electrical permittivity of ultrapure water at the water platinum interface. Chemical physics letters. 2014;613:19–23. [PMC free article] [PubMed] [Google Scholar]
126. Sommer AP, Caron A, Fecht HJ. Tuning nanoscopic water layers on hydrophobic and hydrophilic surfaces with laser light. Langmuir. 2008;24(3):635–636. [PubMed] [Google Scholar]
127. Sommer AP, et al. Pulsed laser light forces cancer cells to absorb anticancer drugs the role of water in nanomedicine. Artificial Cells, Blood Substitutes, and Biotechnology. 2011;39(3):169–173. [PubMed] [Google Scholar]
128. Sommer AP, Haddad MK, Fecht HJ. Light effect on water viscosity: implication for ATP biosynthesis. Scientific reports. 2015;5 [PMC free article] [PubMed] [Google Scholar]
129. Santana-Blank L, et al. “Quantum Leap” in Photobiomodulation Therapy Ushers in a New Generation of Light-Based Treatments for Cancer and Other Complex Diseases: Perspective and Mini-Review. Photomedicine and laser surgery. 2016;34(3):93–101. [PMC free article] [PubMed] [Google Scholar]
130. Santana-Blank L, Rodríguez-Santana E, Santana-Rodríguez KE. Photobiomodulation of aqueous interfaces as selective rechargeable bio-batteries in complex diseases: personal view. Photomedicine and laser surgery. 2012;30(5):242–249. [PubMed] [Google Scholar]
131. Nasouri B, Murphy TE, Berberoglu H. Simulation of laser propagation through a three-layer human skin model in the spectral range from 1000 to 1900 nm. Journal of Biomedical Optics. 2014;19(7):075003–075003. [PubMed] [Google Scholar]
132. Santana-Blank L, Rodriguez-Santana E, Santana-Rodriguez K. Theoretic, experimental, clinical bases of the water oscillator hypothesis in near-infrared photobiomodulation. Photomed Laser Surg. 2010;28(Suppl 1):S41–52. [PubMed] [Google Scholar]
133. Rohani M, Pollack GH. Flow through Horizontal Tubes Submerged in Water in the Absence of a Pressure Gradient: Mechanistic Considerations. Langmuir. 2013;29(22):6556–6561. [PubMed] [Google Scholar]
134. Johnstone DM, et al. Turning On Lights to Stop Neurodegeneration: The Potential of Near Infrared Light Therapy in Alzheimer's and Parkinson's Disease. Frontiers in Neuroscience. 2016;9(500) [PMC free article] [PubMed] [Google Scholar]
135. Santana-Blank L, et al. Water Aos many roles in laser photobiomodulation. Journal of Cancer Research and Treatment. 2015;3(1):1–5. [Google Scholar]
136. Santana-Blank LA, et al. Photo-induced cytomorphologic changes in an advanced cancer phase I clinical trial. Lasers in Surgery and Medicine. 2002;30(1):18–25. [PubMed] [Google Scholar]