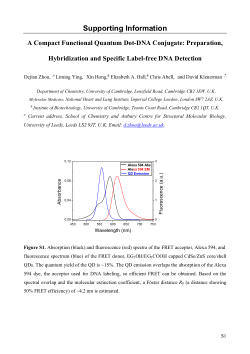
A compact functional quantum dot− DNA conjugate: preparation, hybridization, and specific label-free DNA detection
#WakeUp
#ItsAllFake
#ThereIsNoVirus
😈💩👎
Published article:
Zhou, DJ, Ying, LM, Hong, X, Hall, EA, Abell, C and Klenerman, D (2008) A
Compact Functional Quantum Dot-DNA Conjugate: Preparation, Hybridization
and Specific Label-free DNA Detection. Langmuir, 24 (5). 1659 - 1664. ISSN
0743-7463
http://dx.doi.org/10.1021/la703583u
A Compact Functional Quantum Dot-DNA Conjugate:
Preparation, Hybridization, and Specific Label-Free DNA Detection
snippet "our development of such a signal-on
approach with a compact, covalently coupled QD-DNA
conjugate, where the capturing DNA is coupled to the QD surface
via a tri(ethylene glycol) linker to resist DNA nonspecific
adsorption. We show this system is suitable for both label and
label-free detection of specific DNA at low DNA probe/QD
copy numbers with a sensitivity of ∼1 nM on a conventional"
Dejian Zhou,†,| Liming Ying,‡ Xin Hong,§ Elizabeth A. Hall,§ Chris Abell,† and
David Klenerman*,†
Department of Chemistry, UniVersity of Cambridge, Lensfield Road, Cambridge CB2 1EW,
United Kingdom, Molecular Medicine, National Heart and Lung Institute, Imperial College London,
London SW7 2AZ, United Kingdom, and Institute of Biotechnology, UniVersity of Cambridge,
Tennis Court Road, Cambridge CB2 1QT, United Kingdom
ReceiVed NoVember 16, 2007. In Final Form: January 2, 2008
In this letter, we report the preparation of a compact, functional quantum dot (QD)-DNA conjugate, where the
capturing target DNA is directly and covalently coupled to the QD surface. This enables control of the separation
distance between the QD donor and dye acceptor to within the range of the Fo¨rster radius. Moreover, a tri(ethylene
glycol) linker is introduced to the QD surface coating to effectively eliminate the strong, nonspecific adsorption of
DNA on the QD surface. As a result, this QD-DNA conjugate hybridizes specifically to its complementary DNA
with a hybridization rate constant comparable to that of free DNAs in solution. We show this system is capable of
specific detection of nanomolar unlabeled complimentary DNA at low DNA probe/QD copy numbers via a “signal-on”
fluorescence resonance energy transfer (FRET) response.
Introduction
The unique size-dependent, narrow, symmetric, bright, and
stable fluorescence of quantum dots (QDs) have made them
powerful tools for studying a wide range of biological problems,
from biological imaging and cell tracking and trafficking to novel
multiplexed sensors.1 The broad absorption and narrow emission
spectra of the QDs make them excellent donors in fluorescence
resonance energy transfer (FRET)-based sensors, because these
fluorescence characteristics allow the selection of a wide range
of excitation wavelengths to reduce dye (acceptor) direct
excitation, and proper narrow bandpass filters for the effective
separation of the donor and acceptor fluorescence.2-4 To date,
many of the QD FRET-based sensors have used protein-QD
conjugates, for example, the maltose binding protein (MBP)-
QD3 or the streptavidin-QD4 systems. The capturing biomolecule
is often linked to the QD (mostly CdSe/ZnS core/shell-based)
via a relatively large linker protein, that is, streptavidin.4 The
overall size of the QD donor is made up of the QD core/shell
nanocrystal, and the surface capping and bioconjugation. The
radius of the core/shell nanocrystal determines the color of
fluorescence emission, which varies from ∼1 nm for a 520 nm
QD to 2.6 nm for a 620 nm QD.5 The thickness of the surface
capping and bioconjugation can vary significantly, from 0.5 to
15 nm, depending on the capping methods. A typical Fo¨rster
distance R0, a distance that produces 50% FRET efficiency, for
the QD (donor)-dye (acceptor) FRET systems is between 4-7
nm.2-4 Since the FRET efficiency strongly depends on the donoracceptor distance, it is important to develop a compact QDbiomolecule conjugate to reduce the FRET distance.
Currently, most water-soluble QDs are based on either
wrapping with an amphiphilic polymer or encapping within a
lipid micelle. These produce highly stable water-soluble QDs.
However, these QDs are relatively large, with a hydrodynamic
radius typically >10 nm, greater than the R0 for most QD-dye
FRET systems, even before bioconjugation.5 The relatively large
size of the water-soluble QD and the linker protein is a limiting
factor that often leads to low FRET efficiencies, especially at
low target/QD copy numbers. Consequently, a high copy number
of the target to each QD (usually >10) is required to achieve
high FRET efficiency.4 For example, at a 1:1 target DNA/QD
ratio, Hohng and Ha found that the FRET efficiency between a
streptavidin-coated 585 nm QD and Cy5-labeled DNA acceptor
is very low, with most species being <5.5%.4c However, Wang
and coauthors showed that highly efficient FRET (∼90%) can
be achieved by increasing the copy number of the DNA probe
per QD to 54 using a streptavidin-coated 605 nm QD and Cy5-
labeled DNA.4aTo make highly sensitive QD FRET-based sensors
suitable for low target/QD copy numbers (i.e., 1:1), it is important
to reduce the size of the QD donor. This can be achieved by
reducing the thickness of the QD surface coating, because this
* To whom correspondence should be addressed. Telephone: +44-1223-
336491. Fax: +44-1223-336 362. E-mail: dk10012@cam.ac.uk.
† Department of Chemistry, University of Cambridge.
‡ Imperial College London.
§ Institute of Biotechnology, University of Cambridge.
| Current address: School of Chemistry and Astbury Centre for Structural
Molecular Biology, University of Leeds, Leeds LS2 9JT, United Kingdom.
E-mail: d.zhou@leeds.ac.uk.
(1) (a) Alivisatos, P. Nat. Biotechnol. 2004, 22, 47. (b) Michalet, X.; Pinaud,
F. F.; Bentolila, L. A.; Tsay, J. M.; Doose, S.; Li, J. J.; Sundaresan, G.; Wu, A.
W.; Gambhir, S. S.; Weiss, S. Science 2005, 307, 538. (c) Han, M.-Y.; Gao, X.;
Su, J. Z.; Nie, S. Nat. Biotechnol. 2001, 19, 631. (d) Chan, W. C. W.; Nie, S.
Science 1998, 281, 2016. (e) Klostranec, J. M.; Chan, W. C. W. AdV. Mater. 2006,
18, 1953.
(2) (a) Clapp, A. R.; Medintz, I. L.; Mattoussi, H. ChemPhysChem 2006, 7,
47. (b) Clapp, A. R.; Medintz, I. L.; Mauro, J. M.; Fisher, B. R.; Bawendi, M.
G.; Mattoussi, H. J. Am. Chem. Soc. 2004, 126, 301. (c) Shi, L.; Paoli, V. D.;
Rosenzweig, N.; Rosenzweig, Z. J. Am. Chem. Soc. 2006, 128, 10378. (d) Bakalova,
B.; Zhelev, Z.; Ohba, H.; Baba, Y. J. Am. Chem. Soc. 2005, 127, 11328. (e)
Ruedas-Rama, M. J.; Wang, X.; Hall, E. A. H. Chem. Commun. 2007, 1544. (f)
Potapova, I.; Mruk, R.; Hu¨bner, C.; Zentel, R.; Basche´, T.; Mews, A. Angew.
Chem., Int. Ed. 2005, 44, 2437. (g) Gueroui, Z.; Libchaber, A. Phys. ReV. Lett.
2004, 93, 166108. (h) Lee, S. F.; Osborne, M. J. Am. Chem. Soc. 2007, 129, 8936.
(i) Medintz, I. L.; Berti, L.; Pons, T.; Grimes, A. F.; English, D. S.; Alessandrini,
A.; Facci, P.; Mattoussi, H. Nano Lett. 2007, 7, 1741.
(3) (a) Medintz, I. L.; Clapp, A. R.; Mattoussi, H.; Goldman, E. R.; Fisher,
B.; Mauro, J. M. Nat. Mater. 2003, 2, 630. (b) Medintz, I. L.; Konnert, J. H.;
Clapp, A. R.; Stanish, I.; Twigg, M. E.; Mattoussi, H.; Mauro, J. M.; Deschamps,
J. R. Proc. Natl. Acad. Sci. U.S.A. 2004, 101, 9612. (c) Pons, T.; Medintz, I. L.;
Wang, X.; English, D. S.; Mattoussi, H. J. Am. Chem. Soc. 2006, 128, 15324.
(4) (a) Zhang, C. Y.; Yeh, H. C.; Kuroki, M. T.; Wang, T. H. Nat. Mater. 2005,
4, 826. (b) Oh, E.; Hong, M. Y.; Lee, D.; Nam, S. H.; Yoon, H. C.; Kim, H. S.
J. Am. Chem. Soc. 2005, 127, 3270. (c) Hohng, S.; Ha, T. ChemPhysChem 2005,
6, 956. (d) Levy, M.; Cater, S. F.; Ellington, A. D. ChemBioChem 2005, 6, 2163.
(5) Product specification for Evidots nanomaterials and Evitags from Evident
Technologies Inc. See http://www.evidenttech.com/nanomaterials/evidots/
quantum-dot-research-materials.php.
Langmuir 2008, 24, 1659-1664 1659
10.1021/la703583u CCC: $40.75 © 2008 American Chemical Society
Published on Web 01/15/2008
is where most of the overall QD donor size comes from. In this
regard, functional thiols are very useful because QDs have high
affinity for thiols, and a range of functional hydrophilic groups
can be introduced to the QD surface to achieve water solubility.
Furthermore, the size of the resulting water-soluble QD can be
tailored by controlling the length of the linker between the thiol
and functional terminal group.6,7 We have recently shown that
direct coupling of thiolated fluorophore-labeled double-stranded
DNA to the QD reduces the donor-acceptor distance and
significantly enhances the FRET efficiency especially at low
DNA/QD copy numbers (i.e., 1:1).8 However, to date, specific
detection of target DNA via QD-sensitized FRET in covalently
coupled QD-DNA systems where the QD is capped with
functional thiols has not been demonstrated. This is presumably
because DNAs can strongly, nonspecifically, and irreversibly
adsorb on the QD surface in competition with specific hybridization.7,8 The use of labeled DNAs adsorbed on the QD surface
mediated by a positively charged polymer and FRET signal
reduction in response to specific hybridization have recently
been reported.9 However, this is a signal-off approach. A signalon approach that eliminates nonspecific DNA adsorption should
be more useful because it can potentially offer higher sensitivity.
In this letter, we report our development of such a signal-on
approach with a compact, covalently coupled QD-DNA
conjugate, where the capturing DNA is coupled to the QD surface
via a tri(ethylene glycol) linker to resist DNA nonspecific
adsorption. We show this system is suitable for both label and
label-free detection of specific DNA at low DNA probe/QD
copy numbers with a sensitivity of ∼1 nM on a conventional
fluorimeter.
Experimental Section
Materials. All chemicals, includingN-(3-dimethylaminopropyl)-
N-ethylcarbodiimide hydrochloride (EDC, 99%), N-hydroxysuccinimide (NHS, 98%), sodium bicarbonate, DNAse- and RNAsefree NaCl, high-performance liquid chromatography (HPLC) grade
ethanol, methanol, and chloroform, were purchased from SigmaAldrich (Dorset, U.K.) and used as received unless otherwise stated.
Trioctylphosphine oxide-capped CdSe/ZnS core/shell QDs (TOPOQD, crystal diameter∼2.6 nm, 1.3 mg/mL in toluene) were purchased
from Evident Technologies (Troy, NY). The QD emission peaks at
553 nm with a quantum yield of ∼30%. All DNAs employed in this
study were purchased from IBA GmbH (Go¨ttingen, Germany) and
used as received unless otherwise stated. The DNA sequences and
their abbreviations are given in Table 1. The 5′-C6-amine-modified
capture target strand (DNA-T) and unlabeled DNA probe (DNA-C)
are of HPLC grade, and the Alexa 594-labeled DNAs were double
HPLC purified by the supplier. PBS buffer (10×; 100 mM phosphate,
1.50 M NaCl, 10 mM NaN3, pH 7.2), phosphate buffer (20 mM
phosphate, pH 6.0), and sodium bicarbonate buffer (50 mM NaHCO3,
pH 9.0) were prepared with ultrapure MilliQ water (resistance > 18
MΩ cm). Unless otherwise stated, water means MilliQ water in this
paper.
11-Mercaptoundecyl tri(ethylene glycol) alcohol (EG3OH) was
synthesized following a literature method.10 The crude compound
was purified using flash column chromatography on silica (10%
ethanol in ethyl acetate) to yield the final product (EG3OH) as a
colorless oil. 1H NMR (250 MHz, CDCl3, δ ppm): 1.20-1.37 (m,
14H, 7CH2), 1.49-1.60 (m, 4H, 2CH2), 2.46 (q, 2H, J ) 7.0 Hz,
HSCH2-), 3.05 (s, br, 1H, -OH), 3.40 (t, 2H, J ) 7.0 Hz, -CH2-
EG), 3.50-3.75 (m, 12H, 3(OCH2CH2)). 13C NMR (62.5 MHz,
CDCl3, δ ppm): 72.5, 71.4, 70.5, 70.3, 69.9, 61.5, 34.0, 33.7, 30.5,
29.5, 29.4, 29.0, 28.8, 28.7, 28.3, 26.0, 24.5. HRMS (Q-TOF, ES+):
found, 359.2222; required for C17H36O4SNa [M + Na]+, 359.2232.
11-Mercaptoundecyl tri(ethylene glycol) acetic acid (EG3COOH)
was synthesized following a literature procedure as shown schematically in Scheme 1.11 A modification of the last step was used, where
both protection groups, the thioacetate and t-butyl carboxylic acid
ester, were simultaneously removed in a single step in 0.1 M HCl
in a mixed aqueous/methanol solution heated at reflux. This reduced
the synthesis by one step and more importantly improved the overall
yield of the final product. The crude compound was purified using
flash column chromatography on silica (10% ethanol in ethyl acetate)
to yield the final product as a colorless oil. 1H NMR (250 MHz,
CDCl3, δ ppm): 1.10-1.30 (m, 14H, 7CH2), 1.40-1.52 (m, 4H,
2CH2), 2.40 (q, J ) 7.3 Hz, 2H, HSCH2-), 3.33 (t, J ) 6.9 Hz, 2H,
-CH2-EG), 3.44-3.64 (m, 12H, 3(EG)), 4.06 (s, 2H,-CH2COOH). 13C NMR (100 MHz, CDCl3): 170.8 (CdO), 71.4, 70.9, 70.6, 70.0,
68.6, 51.7, 34.0, 29.6, 29.4, 29.0, 28.3, 26.0, 24.6. HRMS (TOF
ES-): found, 393.2326; required for C19H37O6S [M-H]-, 393.2312.
EG3OH/EG3COOH-capped QDs were prepared by a ligand
exchange procedure as outlined below.12 First, the trioctylphosphineoxide (TOPO)-QD was precipitated by adding ∼1 mL of the
(6) (a) Pathak, S.; Choi, S.-K.; Arnheim, N.; Thompson, M. E. J. Am. Chem.
Soc. 2001, 123, 4103. (b) Uyeda, H. T.; Medintz, I. L.; Jaiswal, J. K.; Simon, S.
M.; Mattoussi, H. J. Am. Chem. Soc. 2005, 127, 3870. (c) Wang, Q.; Xu, Y.; Zhao,
X.; Chang, Y.; Liu, Y.; Jiang, L.; Sharma, J.; Seo, D.-K.; Yan, H. J. Am. Chem.
Soc. 2007, 129, 6380. (d) Susumu, K.; Uyeda, H. T.; Medintz, I. L.; Thomas, P.;
Delehanty, J. B.; Mattoussi, H. J. Am. Chem. Soc. 2007, 129, 13987. (e) Wang,
Q.; Liu, Y.; Ke, Y.; Yan, H. Angew. Chem., Int Ed. 2008, 47, 316.
(7) Zhou, D. J.; Piper, J. P.; Abell. C.; Klenerman, D.; Kang, D. J.; Ying, L.
M. Chem. Commun. 2005, 4807.
(8) Algar, W. R.; Krull, U. J. Langmuir 2006, 22, 11346.
(9) Peng, H.; Zhang, L.; Kjallman, T. H. M.; Soeller, C.; Travas-Sejdic, J. J.
Am. Chem. Soc. 2007, 129, 3048.
(10) (a) Prime, K. L.; Whitesides, G. M. J. Am. Chem. Soc. 1993, 115, 10714.
(b) Zhou, D.; Bruckbauer, A.; Ying, L. M.; Abell, C.; Klenerman, D. Nano Lett.
2003, 3, 1510. (c) Zhou, D. J.; Wang, X. Z.; Birch, L.; Rayment, T.; Abell, C.
Langmuir 2003, 19, 10557.
(11) Roberts, C.; Chen, C. S.; Mrksich, M.; Martichonok, V.; Ingber, D. E.;
Whitesides, G. M. J. Am. Chem. Soc. 1998, 120, 6548.
Table 1. DNA Sequences and Their Abbreviations Used in This Study
DNA code sequence
DNA-T H2NC6H12-5′-CAT AAA AGA GCT CCA TAT CCA ACC TGC ACG-3′
DNA-1 Alexa 594-3′-GTA TTT TCT CGA GGT ATA GGT TGG ACG TGC-5′
DNA-C 3′-GTA TTT TCT CGA GGT ATA GGT TGG ACG TGC-5′
DNA-NC 3′-AAT CAG GGA TTT ACG TGC ACG ACA CAC ACT-5′-Alexa 594
Scheme 1. Synthetic Route to EG3COOH
1660 Langmuir, Vol. 24, No. 5, 2008 Letters
stock toluene solution to ∼10 mL of ethanol, and the resulting
suspension was centrifuged at 14 000 rpm for 5 min. The clear
supernatant was discarded. The pellet was dissolved in ∼0.5 mL of
toluene and precipitated by ethanol, followed by centrifugation. The
clear supernatant was discarded. This process was repeated twice
to remove uncapped free TOPO ligands that could interfere with the
ligand exchange reaction. The pellet was finally dissolved in 1 mL
of CHCl3 and transferred to a 50 mL round-bottom flask, into which
3 mL of ethanol solution of a 2:1 mixture of the EG3OH/EG3COOH
(total thiol concentration 50 mM) and tetramethylammonium
hydroxide (1.3 mol equiv to the total amount of thiols) in methanol
was added. The resulting solution was heated at reflux for 4 h under
a N2 atmosphere. After the solution was cooled to room temperature,
the solvent was removed under reduced pressure. The residue was
dissolved in ∼0.5 mL of ethanol, and CHCl3 (∼5 mL) was added
to precipitate the EG3OH/EG3COOH-capped QDs. The resulting
suspension was centrifuged at 14 000 rpm for 10 min, and the clear
supernatant was discarded. The pellet was then dissolved in ethanol
and precipitated by CHCl3, followed by centrifugation, discarding
the clear supernatant. The process was repeated three times to remove
any uncapped free thiols. Finally, the pellet was dissolved in ∼2 mL
of EtOH/water (1:1, v/v) to obtain the stock solution. The
concentration of the QDs was calculated using the absorbance at the
first exciton peak and the extinction coefficient of 98 000 M-1 cm-1
provided by the manufacturer.
Preparation of the QD-DNA-T Conjugate. EDC (30 mg) and
NHS (15 mg) were dissolved in 200 µL of 1:1 (v/v) EtOH/H2O
mixture, into which 200 µL of phosphate buffer (20 mM, pH 6.0)
was added and thoroughly mixed. After that, 250 µL of the EG3-
OH/EG3COOH-capped QDs (4 µM in water) was added and
thoroughly mixed, and the solution was sonicated in an ultrasonication
bath for 20 s and then allowed to stand at room temperature for 1
h. The solution was then centrifuged at 14 000 rpm for 10 min, and
the clear supernatant (checked by a UV lamp) was discarded. The
red pellet was carefully washed with water (2 × 200 µL) to remove
any residual unreacted EDC/NHS. The pellet was then added to 175
µL of MeOH/H2O (1:1), followed by 25 µL of C6-amine-modified
DNA-T (440 µM, the ratio of DNA-T/QD ) 11:1) and 100 µL of
NaHCO3 buffer (50 mM, pH 9.0). The mixture was sonicated for
2 min to break up the pellet, and then the solution was stored at
4 °C overnight to obtain a slightly brownish solution. The sample
was centrifuged at 14 000 rpm for 20 min, and the clear supernatant
was carefully separated from the pellet. The pellet was washed with
200 µL of MeOH/H2O (1:1). A UV-vis absorption spectrum was
taken on the combined supernatant, and the absorbance at 260 nm
was used for the calculation of the amount of DNAs not conjugated
to the QD using a molecular extinction coefficient of 292 000 M-1
cm-1 for DNA-T, as provided by the manufacturer (the supernatant
was first checked with a UV lamp to ensure it was free of QDs,
otherwise the strong absorption of the QDs at 260 nm will interfere
with the measurement). The number of DNA-T attached to each QD
was estimated to be 2.2, and thus, a coupling efficiency of 20% was
obtained based on a starting DNA-T/QD ratio of 11. The pellet was
added to 0.5 mL of pure water, sonicated for 5 min to obtain a clear
stock solution, and stored in the dark at 4 °C until use.
Hybridization of Probe DNAs with the QD-DNA-T Conjugate. The total volume of the hybridization reaction solution was
kept constant at 500 µL, with a final QD concentration of 100 nM
in 1× PBS. The reaction was carried out in batches under identical
conditions, where 50 µL of 10× PBS, the calculated amount of pure
water, (ethidium bromide where necessary), DNA probe (this varies
in different DNA probe/QD ratios), and the required amount of the
QD-DNA-T conjugate were sequentially added to a series of
Eppendorf tubes and thoroughly mixed on a vortex mixer. The
hybridization reaction was carried out at room temperature for 2 h
before fluorescence spectra were taken. For kinetic studies, the
required QD-DNA-T conjugate solution in 1× PBS was prepared
and transferred to a fluorescence cuvette, and a fluorescence spectrum
was taken. A calculated amount of DNA-1 was then added and
quickly mixed with a micropipet. Fluorescence spectra were then
recorded at different reaction intervals to obtain kinetic information.
The dilution of the QD-DNA-T conjugate resulting from the
increased volume following the addition of DNA-1 was corrected
for.
UV-Vis Spectra. The absorption spectra of the QD-DNA
conjugates were measured on a Cary 300 Bio UV-vis spectrophotometer (Varian Inc., CA). A spectral range of 220-800 nm was
recorded at a scan rate 600 nm/min at a slit width of 2 nm with a
quartz cuvette. The spectral background was corrected with blank
1× PBS buffer using the same cuvette.
Fluorescence Spectra. All fluorescence spectra were recorded
on an Aminco-Bowman Series 2 Luminescence spectrometer (SimAminco Spectronic Instruments Inc, Rochester, NY).5 The emission
spectra (500-800 nm range) were recorded under a fixed excitation
wavelength of 445 nm (to minimize direct excitation of the Alexa
594 dye) at a scan rate of 2 nm/s. An excitation and emission
bandwidth of 4 nm was used. For the QD-DNA conjugates, where
the DNA was labeled with the Alexa 594 fluorophore, the
fluorescence spectra were corrected for direct excitation of the dye
by using the same dye-labeled DNA as reference. The quantum
yield of the QD was measured using Rhodamine-6G in ethanol
(95% under 480 nm excitation) as a reference. The optical densities
of the QD and Rhodamine-6G solutions used were 0.05 at 480 nm.
Since the fluorescence quantum yield of QDs in the QD-DNA
conjugates is dependent on the number of DNAs attached to each
QD, the approximate FRET efficiency was estimated using E )
IA/(IA + ID), where ID and IA are donor and acceptor fluorescence
intensities, respectively, rather than using donor quenching.
Calculation of the FRET Signal. The fluorescence intensity
referred to in this paper is the integrated fluorescence. The Alexa
594 fluorescence from the hybridized sample was obtained using
the integrated fluorescence of the whole spectrum (after correction
for direct excitation of Alexa 594) after subtracting the QD
fluorescence. The calculation was carried out assuming that the QD
fluorescence maintained the same shape as that of the QD-DNA-T
conjugate only sample, so its integrated fluorescence is proportional
to the height of the QD fluorescence peak (Alexa 594 does not
fluoresce at the QD fluorescence peak, 558 nm).
Fitting of the Hybridization Kinetic Data. Two assumptions
have been used to fit the kinetic data: (1) the FRET signal arises
from the 1:1 hybridized QD-DNA-1 conjugate and (2) the maximum
FRET signal corresponding to a QD-DNA-T/DNA-1 conjugate
concentration equals the QD starting concentration. This assumption
is needed to estimate the percentage of the QD-DNA-T conjugate
hybridized. These assumptions are reasonable because the hybridization reactions were carried out at concentrations much higher
than the dissociation constant (Kd < 1 nM) of a 30-mer duplex
DNA. The hybridization reaction can be described as
where the starting concentrations for the QD-DNA-T and DNA-1
are the same, CQD ) CDNA-1 ) C0.
So, the rate of the hybridization reaction can be described by
second-order kinetics as
where kA is the hybridization rate constant and Cis the concentration
of the QD-DNA-T/DNA-1 conjugate. Thus
At the beginning of the hybridization reaction, when t ) 0, C )
0. Integration of the equation gives (12) (a) Wuister, S. F.; Swart, I.; van Driel, F.; Hickey, S. G.; Donega, C. D.
Nano Lett. 2003, 3, 503. (b) Zhou, D. J.; Bruckbauer, A.; Abell, C.; Klenerman,
D.; Kang, D.-J. AdV. Mater. 2005, 17, 1243.
QD-DNA-T + DNA-1 f QD-DNA-T/DNA-1 (1)
dC/dt ) kACQDCDNA-1 ) kA[C0 - C]
2 (2)
dC/[C0 - C]
2 ) kA dt (3)
C/C0 ) 1 - 1/(C0kAt + 1) ) 1 - 1/(kt + 1) (4)
Letters Langmuir, Vol. 24, No. 5, 2008 1661
where k)C0.kA. Since the fluorescence of the QD-DNA-T conjugate
can gradually degrade when exposed to PBS, a factor for this
correction is also added (assuming the degradation is linear with
incubation time). Thus, the equation used to fit the curve is
All the kinetic curves were fitted using this equation. The value of
kA was obtained from the fitting parameter of k divided by C0, the
starting concentration.
Results and Discussion
Figure 1 shows our approach schematically. The commercial
trioctylphosphineoxide (TOPO)-capped CdSe/ZnS core/shell QDs
(emission peak ∼ 553 nm, quantum yield ∼ 30%) are made
water-soluble by ligand exchange with a 2:1 mixture of EG3-
OH/EG3COOH in mixed solvents of chloroform/ethanol. This
produces stable, water-soluble EG3OH/EG3COOH-capped QDs,
where the functional hydrophilic hydroxyl (OH) and carboxylic
acid (COOH) terminal groups are spaced out from the QD surface
with a tri(ethylene glycol) (EG3) spacer. The introduction of the
EG3 group to the surface capping enhances the stability and
solubility of the QD in aqueous media6d and more importantly
provides a surface coating that resists the nonspecific adsorption
of DNAs.11 The functional QD-DNA conjugate was prepared
by first activation of the EG3OH/EG3COOH-capped QD with
EDC/NHS in phosphate buffer (pH 6.0), followed by coupling
of the 5′-C6-amine-modified 30-mer target DNA (DNA-T) to
the QD surface carboxylate group via the formation of an amide
linker in sodium bicarbonate buffer (pH 9.0). Hybridization of
a fluorophore (Alexa 594)-labeled DNA complementary to
DNA-T brings the fluorophore in close proximity to the QD, so
when the QD is excited, it efficiently undergoes energy transfer
to the fluorophore via FRET, producing a dye fluorescence signal
that can be used for detection of the labeled complementary
DNA probe (route A). Noncomplementary probes do not hybridize
to the QD-DNA-T conjugate, so they do not participate in the
FRET process and are therefore nonfluorescent. This makes the
removal of such probes unnecessary, a distinct advantage for the
FRET-based system. Alternatively, an unlabeled complementary
DNA and ethidium bromide (EB), a dye known to specifically
intercalate double-stranded DNAs,14 are simultaneously introduced to the system. The formation of duplex DNA via
hybridization leads to EB intercalation,14 so excitation of the QD
leads to energy transfer from the QD to EB, producing EB
fluorescence that can be used for detection and quantification of
unlabeled DNAs (route B). This should be more useful from the
sensor application point of view, because it does not require
labeling of the probes. Noncomplementary probes will not produce
any EB FRET signal because they cannot hybridize to the QDDNA-T conjugate, and so no EB will intercalate.
To make a highly water-soluble EG3OH/EG3COOH-capped
QD, it is important to remove the free TOPOs in the QD stock
solution, otherwise incomplete ligand exchange will produce a
QD of poor aqueous solubility. The water-soluble EG3OH/EG3-
COOH-capped QD prepared in this study was stable for at least
1 month when stored in the dark at 4 °C. No changes in the
fluorescence intensity and spectral shape were observed within
this period. It maintains a sharp emission band (fwhm ∼ 30 nm)
similar to that of the original TOPO-QD, with a slight red-shift
of the emission peak to 558 nm. The quantum yield is ∼15%,
about half that of the original TOPO-QD, but this still compares
favorably to most of the commercial water-soluble QDs.7 The
QD emission significantly overlaps the absorption of the Alexa
594 fluorophore, the acceptor used for DNA labeling, so efficient
FRET can be obtained in this system (Supporting Information,
Figure S1). Based on the spectral overlap and the molecular
extinction coefficient of Alexa 594, a Fo¨ster distance R0 of ∼4.2
nm is estimated for this QD-Alexa 594 FRET system.7 On
average, there are 2.2 DNA-T molecules attached to each QD
in our QD-DNA-T conjugate prepared in this study.
Hybridization of complementary DNA-1 (labeled with Alexa
594 at 3′; see Table 1) to the QD-DNA-T conjugate was carried
out using 100 nM QD in 1× PBS (10 mM phosphate, 150 mM
NaCl, pH 7.2) at different DNA-1/QD ratios. The corresponding
fluorescence spectra (all corrected for background from direct
dye excitation) are shown in Figure 2A. It is clear that
hybridization of DNA-1 to the QD-DNA-T conjugate quenches
the QD emission at 558 nm while enhancing the emission of
Alexa 594 at 618 nm via FRET. The apparent FRET efficiency,
E ) IA/(IA + ID), where IA and ID are the integrated acceptor
(Alexa 594) and donor (QD) fluorescence (see Experimental
Section for details),2,3,7 increases approximately linearly with
the increasing copy numbers of DNA-1 per QD initially, and
then it levels off at a ratio of just over 2 (Figure 2B). This is in
excellent agreement with the estimation that there are only 2.2
DNA-Ts attached to each QD. This confirms that all the DNA-T
molecules coupled to the QD are functional and available for
hybridization. We found that the use of PBS is crucial to achieving
specific hybridization. Another buffer, such as Tris (10 mM Tris
HCl, 100 mM NaCl, pH 7.6), produced significant nonspecific
DNA adsorption.
To confirm that the observed FRET signal is due to specific
DNA hybridization, and not from nonspecific adsorption, three
control experiments were carried out. (1) The EG3OH/EG3COOHcapped QD (100 nM) without any DNA-Ts attached was mixed
with DNA-1 (220 nM) in 1× PBS under identical conditions.
This did not produce any detectable Alexa 594 FRET signal
(Supporting Information, Figure S2A). This eliminates the
possibility that the FRET signal is due to the nonspecific
adsorption of DNA-1 on the QD surface. (2) The QD-DNA-T
conjugate (100 nM) and DNA-1 (220 nM) were mixed in pure
water without salt. No Alexa 594 FRET signal was detected,
suggesting that no hybridization had taken place. However, upon
addition of 50 mM NaCl (final concentration) to this system, a
significant FRET signal was observed, confirming that DNA
(13) Sekar, M. M. A.; Bloch, W.; Pamela, M.; St John, P. M. Nucleic Acids
Res. 2005, 33, 366.
(14) He, F.; Tang, Y.; Yu, M.; Feng, F.; An, L.; Sun, H.; Wang, S.; Li, Y.;
Zhu, D.; Bazan, G. C. J. Am. Chem. Soc. 2006, 128, 6764.
Figure 1. Schematic representation of the principles of our
approaches for hybridization and label-free detection of DNA probes
with a covalently coupled QD-DNA-T conjugate via a QD sensitized
FRET signal.
C/C0 ) 1 - 1/(kt + 1) - at (5)
1662 Langmuir, Vol. 24, No. 5, 2008 Letters
hybridization did take place (Supporting Information, Figure
S2B). It is well-known that DNA hybridization is salt dependent;
without the salt counterions to shield the strong electrostatic
repulsion from the negatively charged phosphate backbones, the
DNA duplex could not form. The fact that the Alexa 594 FRET
signal is only observed in the presence of moderate salt supports
that the FRET signal is indeed due to DNA hybridization. (3)
Alexa 594-labeled control DNA (DNA-NC, 30-mer but with a
noncomplementary sequence to DNA-T) was incubated with the
QD-DNA-T conjugate. This produced no detectable Alexa 594
FRET signal (Supporting Information, Figure S2C). The three
control experiments confirm unambiguously that the observed
Alexa 594 FRET signal is indeed due to the specific hybridization
between complementary DNAs. This is a significant improvement
in covalently coupled QD-DNA systems, where other systems
lacking the EG3 spacer have exhibited strong nonspecific
adsorption of DNAs,8 suggesting that the introduction of the
EG3 linker to the QD surface coating effectively eliminates the
nonspecific adsorption of DNA on the QD surface. This suggests
that this system is suitable for specific detection of labeled
complementary probes.
Figure 3 shows the time-dependent hybridization induced
FRET signal between the DNA-1 and QD-DNA-T conjugate
at a fixed DNA-1/QD ratio of 1:1 at different concentrations.
Compared with other covalent QD-DNA systems, where a
complete hybridization requires over 8 h,8 our system is ∼50
times faster and is complete in ∼10 min. This suggests that the
DNA-Ts in our system are not significantly hindered and can
readily hybridize. The time-dependent fluorescence can be fitted
to second-order reaction kinetics (See Experimental Section for
details of the fitting). The value of the hybridization rate constant
kA can be obtained from the fitting parameter of k divided by C0,
the starting concentration. The best fitting parameter k is 0.017
( 0.002, 0.0081 ( 0.0003, and 0.0072 ( 0.0002 s-1 for the 100,
50, and 25 nM samples, respectively. This gives a kA value of
1.7 ( 0.2, 1.6 ( 0.1, and 2.9 ( 0.1 × 105 M-1 s-1 for the 100,
50, and 25 nM samples, respectively. These values are comparable
to those of free DNAs without a secondary structure,13 suggesting
that conjugation of DNA-T to the QD surface does not affect the
hybridization kinetics significantly.
Figure 2. (A) Fluorescence spectra of the QD-DNA-T conjugate
after hybridization with DNA-1 at different molar ratios. All
experiments were carried out in 1× PBS with 100 nM QD-DNA-T
conjugate excited at 445 nm. (B) Plot of the apparent FRET efficiency
versus the DNA-1/QD ratios.
Figure 3. Time-dependent FRET signal showing the hybridization
kinetics between QD-DNA-T and DNA-1 at a 1:1 ratio at 100 nM
(solid squares), 50 nM (filled triangles), and 25 nM (filled circles).
The red lines are the fits using a second-order reaction kinetics as
described in the experimental section.
Figure 4. (A) Fluorescence spectra of the QD-DNA-T conjugate
for label-free detection of DNA-C. The spectra were recorded in
PBS excited at 378 nm. (B) A plot of the EB FRET signal (integrated
fluorescence from 575 to 740 nm, background corrected) versus the
concentration of DNA-C.
Letters Langmuir, Vol. 24, No. 5, 2008 1663
Having demonstrated that this QD-DNA-T conjugate is
functional and can be used for specific detection of labeled
complementary probes at low probe/QD copy numbers, a more
important step was to investigate its potential for detecting
unlabeled probes. In this regard, we have explored a strategy
based on the fact that ethidium bromide (EB) specifically
intercalates duplex DNA without sequence specificity, as the
fluorescence readout (Figure 1, route B).14 These experiments
were carried out under the same experimental conditions as before,
where each sample contained 100 nM QD-DNA-T conjugate,
3 µM EB, and different concentrations of unlabeled complementary DNA-C and was allowed to hybridize for 2 h. The
corresponding fluorescence spectra are shown in Figure 4A. It
is clear that the EB fluorescence increases with increasing DNA-C
concentration. Introduction of a noncomplementary unlabeled
DNA (500 nM) to the (QD-DNA-T + EB) system did not
produce any observable EB fluorescence, confirming that the
observed EB FRET signal is DNA sequence specific. This is
presumably because EB binds strongly to double-stranded DNAs
without any sequence specificity,14 whereas, for single-stranded
DNAs, EB binding is much weaker and has some sequence
specificity.15 The fact that no EB fluorescence was detected here
for the QD-DNA-T/EB system in the absence of DNA-C may
suggest that the DNA-T sequence used in this study does not
bind EB or the binding is too weak to be detectable under our
experimental conditions. A plot of the integrated EB FRET signal
versus the concentration of DNA-C shows a very good linear fit
(R ) 0.999, Figure 4B), suggesting that this system is wellsuited for label-free detection and quantification of complementary DNA analyte. The detection limit here is ∼1 nM using
a conventional fluorimeter. This can be improved by using shorter
alkyl linkers between the QD and DNA-T to improve the FRET
efficiency. Even with this by no means optimized system, the
sensitivity achieved here is already better than the recently reported
QD FRET based on a signal-off approach,9 demonstrating the
excellent potential of this signal-on approach.
In summary, we have prepared a compact, covalently coupled
functional QD-DNA conjugate and demonstrated the detection
specific, unlabeled nanomolar complementary DNA via a QDsensitized Alexa 594 FRET signal at low DNA probe/QD copy
numbers. This has been achieved by incorporation of an EG3
linker into the QD surface coating that effectively eliminated the
nonspecific adsorption of DNAs on the QD surface, allowing
specific hybridization of complementary DNA to the QDDNA-T conjugate. Further optimization of this system is currently
under way, to reduce the alkyl linker length of the thiol ligand
and to improve the stability of the water-soluble QD by using
chelating ligands. These developments in combination with the
DNA/RNA aptamers16 will lead to a general, robust, highly
sensitive, and selective QD FRET-based sensing platform suitable
for the detection of a wide range of targets, from important disease
markers and metal ions to drug molecules.
Acknowledgment. This work was supported as part of the
University of Cambridge-ETRI (Korean) Joint International
Research Collaboration.
Supporting Information Available: Supporting figures showing
the absorption and fluorescence spectra of Alexa 594 and their overlapping
with QD fluorescence, and fluorescence spectra of the control experiments. This material is available free of charge via the Internet at
http://www.acs.org.
(15) (a) Sarov-Blat, L.; Livneh, Z. J. Biol. Chem. 1998, 273, 5520. (b) Davies, LA703583U
D. B.; Baranovsky, S. F.; Veselkov, A. N. J. Chem. Soc., Faraday Trans. 1997,
93, 1559. (16) Bunka, D. H. J.; Stockley, P. G. Nat. ReV. Microbiol. 2006, 4, 588.
1664 Langmuir, Vol. 24, No. 5, 2008 Letters